Compendium of Bioenergy Plants: Corn
Total Page:16
File Type:pdf, Size:1020Kb
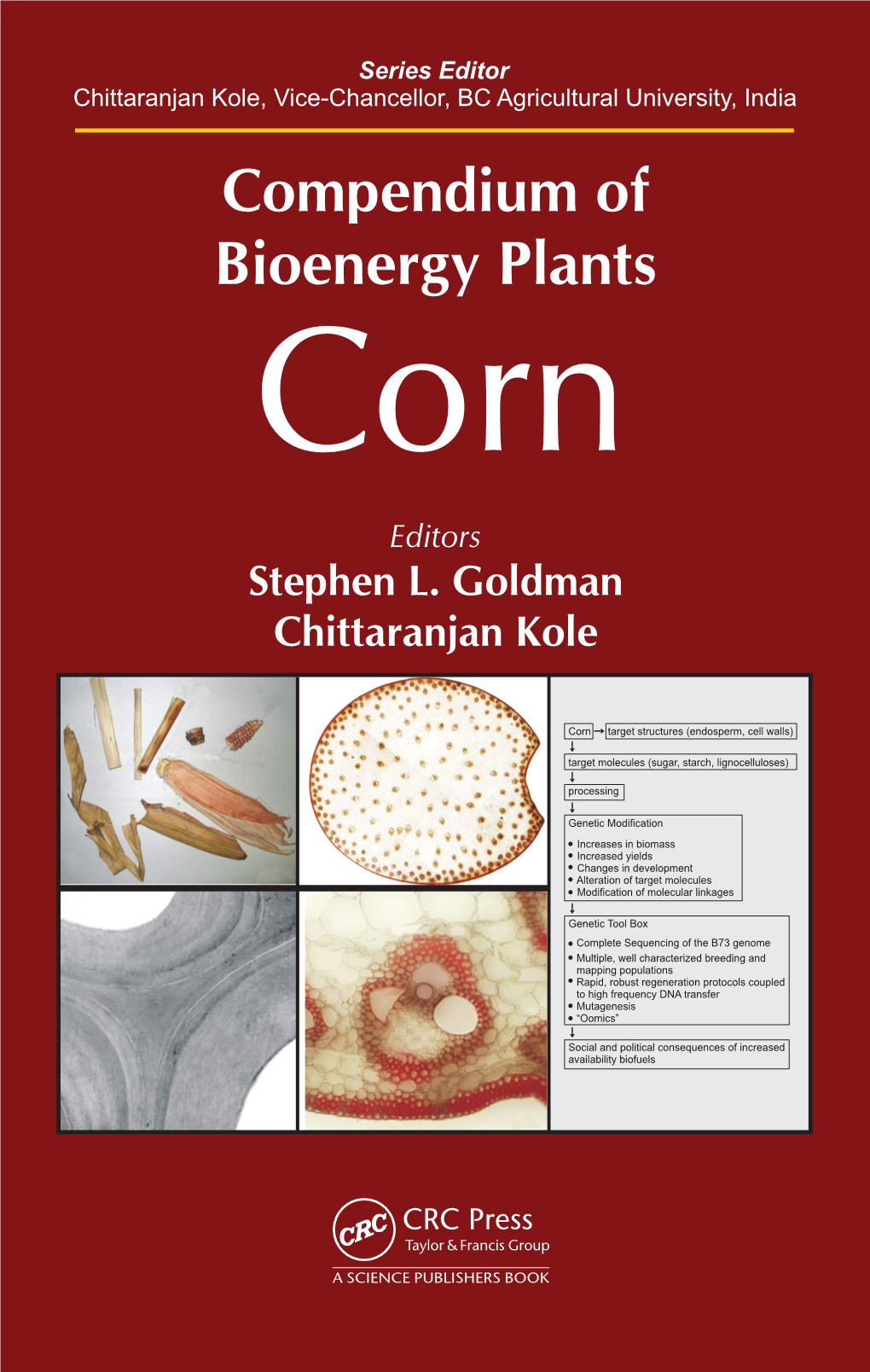
Load more
Recommended publications
-
Role of Starch Structure in Texture of Winter Squash (Cucurbita Maxima D) Fruit and Starch Functional Properties David Graham Stevenson Iowa State University
Iowa State University Capstones, Theses and Retrospective Theses and Dissertations Dissertations 2003 Role of starch structure in texture of winter squash (Cucurbita maxima D) fruit and starch functional properties David Graham Stevenson Iowa State University Follow this and additional works at: https://lib.dr.iastate.edu/rtd Part of the Agriculture Commons, and the Food Science Commons Recommended Citation Stevenson, David Graham, "Role of starch structure in texture of winter squash (Cucurbita maxima D) fruit and starch functional properties " (2003). Retrospective Theses and Dissertations. 1465. https://lib.dr.iastate.edu/rtd/1465 This Dissertation is brought to you for free and open access by the Iowa State University Capstones, Theses and Dissertations at Iowa State University Digital Repository. It has been accepted for inclusion in Retrospective Theses and Dissertations by an authorized administrator of Iowa State University Digital Repository. For more information, please contact [email protected]. Role of starch structure in texture of winter squash (Cucurbita maxima D.) fruit and starch functional properties by David Graham Stevenson A dissertation submitted to the graduate faculty in partial fulfillment of the requirements for the degree of DOCTOR OF PHILOSOPHY Major: Food Science and Technology Program of Study Committee: Jay-lin Jane, Major Professor Pamela White Jane Love John Robyt Ted Bailey Iowa State University Ames, Iowa 2003 UMI Number: 3105108 UMI UMI Microform 3105108 Copyright 2003 by ProQuest Information and Learning Company. All rights reserved. This microform edition is protected against unauthorized copying under Title 17, United States Code. ProQuest Information and Learning Company 300 North Zeeb Road P.O. Box 1346 Ann Arbor, Ml 48106-1346 ii Graduate College Iowa State University This is to certify that the Doctoral dissertation of David Stevenson has met the dissertation requirements of Iowa State University Signature was redacted for privacy. -
The Influence of Starch Modification with Amylosucrase Treatment On
processes Article The Influence of Starch Modification with Amylosucrase Treatment on Morphological Features Hyeyoung Lee 1 and Inmyoung Park 2,* 1 Division of Applied Bioengineering, Dong-eui University, Busan 47340, Korea; [email protected] 2 Division of Food and Culinary Arts, Youngsan University, Busan 48015, Korea * Correspondence: [email protected]; Tel.: +82-51-540-7236 Received: 22 October 2020; Accepted: 3 November 2020; Published: 4 November 2020 Abstract: Amylosucrase (AS) is a starch-modifying enzyme from Neisseria polysaccharea used to produce low-glycemic starches such as slowly digestible starch (SDS) and resistant starch (RS). The morphology of native, control, and AS-modified waxy corn starches (230 and 460 U) was examined using a particle size analyzer and field-emission scanning electron microscopy (FE-SEM). AS modification of the starch elongated the glucose and resulted in higher SDS and RS contents. The mean particle sizes of the control, 230 U-AS-, and 460 U-AS-treated starches were 56.6 µm, 128.0 µm, and 176.5 µm, respectively. The surface of the 460 U-AS-treated starch was entirely porous and coral-like, while the 230 U-AS-treated starch had a partial dense and flat surface which did not react with AS. FE-SEM of the granule cross section confirmed that the center contained a dense and flat region without any evidence of AS reaction to either of the AS-treated starches. It was assumed that the particle size and porous and sponge-like particle features might be related to the SDS and RS fractions. Keywords: amylosucrase-modified starch; particle size distribution; cross-sectioned particle; morphology; low glycemic starch 1. -
Corn Grain Processing and Digestion
CORN GRAIN PROCESSING AND DIGESTION Fred Owens Pioneer Hi-Bred International, Inc., Johnston, IA SUMMARY Grains are fed to livestock primarily to supply energy, and the major energy source in cereal grains is starch. For maximum starch digestion, corn and sorghum grain must be processed. For non-ruminants, starch from finely ground grain is fully digested, but for ruminants fed concentrate diets, finely ground grain can cause metabolic diseases. Hence, steam rolling or flaking and fermentation (high moisture storage) rather than fine grinding are used for grains fed to ruminants to increase the extent of starch digestion. Such processing methods increase starch digestion both in the rumen (of dietary starch) and postruminally (of starch reaching the small intestine). The lower the density of corn flakes, the greater the digestibility of starch, particularly in the small intestine. For maximum ruminal starch digestion, a thinner flake is needed for lactating cows than for feedlot cattle because particles spend less time in the rumen for digestion in lactating cows than in feedlot cattle. This shortened ruminal residence time can explain why ruminal and total tract starch digestibility is lower for lactating cows than for finishing cattle. Contrary to popular belief, digestion of starch reaching the small intestine does not decrease as abomasal supply of starch increases. However, neither dry rolled or whole corn is digested well post-ruminally. Due to reduced loss of methane and heat, available energy supply for the animal is greater for starch digested in the small intestine than for starch fermented in either the rumen or large intestine. Different hybrid characteristics are desired for different processing methods. -
Thermal Characterization of Corn Starch Mutants and Textural Effects on Tortillas Kim Anne Rohlfing Iowa State University
Iowa State University Capstones, Theses and Graduate Theses and Dissertations Dissertations 2009 Thermal Characterization of Corn Starch Mutants and Textural Effects on Tortillas Kim Anne Rohlfing Iowa State University Follow this and additional works at: https://lib.dr.iastate.edu/etd Part of the Nutrition Commons Recommended Citation Rohlfing, Kim Anne, "Thermal Characterization of Corn Starch Mutants and Textural Effects on Tortillas" (2009). Graduate Theses and Dissertations. 10763. https://lib.dr.iastate.edu/etd/10763 This Thesis is brought to you for free and open access by the Iowa State University Capstones, Theses and Dissertations at Iowa State University Digital Repository. It has been accepted for inclusion in Graduate Theses and Dissertations by an authorized administrator of Iowa State University Digital Repository. For more information, please contact [email protected]. Thermal characterization of corn starch mutants and textural effects on tortillas By Kimberly Anne Rohlfing A thesis submitted to the graduate faculty In partial fulfillment of the requirements for the degree of MASTER OF SCIENCE Major: Food Science and Technology Program of Study Committee: Pamela J. White, Major Professor Cheryll A. Reitmeier Linda M. Pollak Iowa State University Ames, Iowa 2009 Copyright © Kimberly Anne Rohlfing, 2009. All rights reserved. ii TABLE OF CONTENTS LIST OF FIGURES iii LIST OF TABLES iv ABSTRACT v CHAPTER 1. GENERAL INTRODUCTION Introduction 1 Literature Review 2 References 38 CHAPTER 2. THERMAL CHARACTERISTICS OF STARCH FROM CORN MUTANTS AND EXOTICS WITH DIFFERENT AMOUNTS OF RESISTANT STARCH Abstract 58 Introduction 59 Materials and Methods 71 Results and Discussion 64 Conclusion 67 References 78 CHAPTER 3. RESISTANT STARCH EFFECTS ON TORTILLA TEXTURE Abstract 79 Introduction 79 Materials and Methods 82 Results and Discussion 86 Conclusion 89 References 89 CHAPTER 4. -
Cereal Grains Structure & Composition Topics of Discussion
Ist US-Brazil Fulbright Course on Biofuels, Sao Singh – Corn Structure and Composition Paulo, Brazil Cereal Grains Structure & Composition Vijay Singh Associate Professor Department of Agricultural & Biological Engineering University of Illinois at Urbana-Champaign, Urbana, IL 1st Brazil-U.S. Biofuels Short Course São Paulo, Brazil July 27 - August 7, 2009 Topics of Discussion • Structure of Corn Kernel – Hand dissection exercise •Starch • Protein •Oil •Fiber • Other Constituents • Corn Composition University of Illinois at Urbana-Champaign Ist US-Brazil Fulbright Course on Biofuels, Sao Singh – Corn Structure and Composition Paulo, Brazil Structure of Cereal Grains • Cereals are members of grass family • Produce dry , one-seeded fruit , called caryopsis • Caryopsis is also called kernel or grain • Caryopsis consists of – Fruit coat or pericarp, which surrounds seed and is tightly adhered to seed coat – Seed, which consists of germ or embryo and endosperm enclosed bllididdby a nucellar epidermis and a seed coat • All cereal grains have these same parts in approx. same relationship to each other Structure of Corn Kernel Pericarp Endosperm Germ Tip Cap University of Illinois at Urbana-Champaign Ist US-Brazil Fulbright Course on Biofuels, Sao Singh – Corn Structure and Composition Paulo, Brazil Scanning Electron Micrograph (SEM) of Dissected Steeped Corn Kernel Pericarp Endosperm Pericarp • 5 to 6% of kernel dry weight Cuticle Epidermis Mesocarp Cross cells Tube cells Seed coat • Cells in pericarp: hollow tubes, channels for water absorption via tip cap • Seed coat: semipermeable • Walls of pericarp contain cellulose and pentosans, no lignin. University of Illinois at Urbana-Champaign Ist US-Brazil Fulbright Course on Biofuels, Sao Singh – Corn Structure and Composition Paulo, Brazil Aleurone layer • Aleurone layer beneath seed coat • Aleurone layer cells contain protein bodies (good amino acid profile), oil bodies, and no starch. -
Yüksek Amilozlu Misir Nişastasindan Dirençli Nişasta Eldesi Ve Erişte Üretiminde Kullanimi
YÜKSEK AMİLOZLU MISIR NİŞASTASINDAN DİRENÇLİ NİŞASTA ELDESİ VE ERİŞTE ÜRETİMİNDE KULLANIMI Ayşe Neslihan (İNKAYA) DÜNDAR YÜKSEK AMİLOZLU MISIR NİŞASTASINDAN DİRENÇLİ NİŞASTA ELDESİ VE ERİŞTE ÜRETİMİNDE KULLANIMI Ayşe Neslihan (İNKAYA) DÜNDAR Ayşe Neslihan (İNKAYA) DÜNDAR T.C. ULUDAĞ ÜNİVERSİTESİ FEN BİLİMLERİ ENSTİTÜSÜ GIDA MÜHENDİSLİĞİ ANABİLİM DALI YÜKSEK AMİLOZLU MISIR NİŞASTASINDAN DİRENÇLİ NİŞASTA ELDESİ ve ERİŞTE ÜRETİMİNDE KULLANIMI Ayşe Neslihan İNKAYA (DÜNDAR) Prof. Dr. Duygu GÖÇMEN (Danışman) DOKTORA TEZİ GIDA MÜHENDİSLİĞİ ANABİLİM DALI BURSA-2014 Her Hakkı Saklıdır TEZ ONAYI Ayşe Neslihan (İNKAYA) DÜNDAR tarafından hazırlanan “Yüksek Amilozlu Mısır Nişastasından Dirençli Nişasta Eldesi ve Erişte Üretiminde Kullanımı” adlı tez çalışması aşağıdaki jüri tarafından oy birliği/oy çokluğu ile Uludağ Üniversitesi Fen Bilimleri Enstitüsü Gıda Mühendisliği Anabilim Dalı’nda DOKTORA TEZİ olarak kabul edilmiştir. Danışman : Prof. Dr. Duygu GÖÇMEN Başkan : Prof.Dr.Duygu GÖÇMEN İmza U.Ü. Ziraat Fakültesi. Gıda Mühendisliği Anabilim Dalı Üye : Prof.Dr. Fikri BAŞOĞLU İmza U.Ü. Ziraat Fakültesi. Gıda Mühendisliği Anabilim Dalı Üye : Doç.Dr. Ramazan DOĞAN İmza U.Ü. Ziraat Fakültesi. Tarla Bitkileri Anabilim Dalı Üye : Doç.Dr. Yasemin ŞAHAN İmza U.Ü. Ziraat Fakültesi. Gıda Mühendisliği Anabilim Dalı Üye : Doç. Dr. Nermin BİLGİÇLİ İmza Necmettin Erbakan Üniversitesi Mimarlık Mühendislik Fakültesi Gıda Mühendisliği Anabilim Dalı Yukarıdaki sonucu onaylarım Prof. Dr. Ali Osman DEMİR Enstitü Müdürü ../../….(Tarih) U.Ü. Fen Bilimleri Enstitüsü, tez -
Origin, Adaptation, and Types of Corn W
NATIONAL CORN HANDBOOK THE CORN CROP NCH-10 Origin, Adaptation, and Types of Corn W. L. Brown, Pioneer Hi-Bred International, Inc., IA; M. S. Zuber, University of MIssouri; L. L. Darrah, USDA-ARS, University of MIssouri; and D. V. Glover, Purdue University Reviewers R. G. Creech, Mississippi State University A. A. Fleming, University of Georgia K. F. Schertz, USDA-ARS, Texas A&M University A. F. Troyer, DeKalb-Pfi zer Genetics, IL ORIGIN OF CORN attention were given to determining corn’s origin and Corn (Zea mays L.) is the only important cereal more to understanding the remarkable variability found indigenous to the Western Hemisphere. Apparently within the species. originating in Mexico, it spread northward to Canada and southward to Argentina. While the possibility of Variability and Races secondary centers of origin in South America cannot Regardless of origin, corn has proven to be one of be completely ruled out, the oldest (7000 years) the most adaptable and variable members of the grass archaeological corn was found in Mexico’s Valley of family. Its evolution, a large part of which apparently Tehuacan. occurred under domestication, has resulted in biotypes The earliest “corn” of which there is record is with adaptation ranging from the tropics to the north unmistakably corn. The female infl orescence of this temperate zone, from sea level to 12,000 feet altitude 5000 B.C. corn had reached a degree of specialization and growing periods (planting to maturity) extending that precluded the possibility of natural seed dissemi- from 6 weeks to 13 months. nation. Thus, the oldest corn of record was dependent Almost 300 races of corn have been described upon man for its survival. -
Structure of Potato Starch
Academic Press is an imprint of Elsevier 30 Corporate Drive, Suite 400 Burlington, MA 0183, USA 525 B Street, Suite 1900, San Diego, CA 92101-4495, USA 32 Jamestown Road, London, NW1 7BY, UK 360 Park Avenue South, New York, NY 10010-1710, USA First edition 2009 Copyright © 2009, Elsevier Inc. All rights reserved No part of this publication may be reproduced, stored in a retrieval system or transmitted in any form or by any means electronic, mechanical, photocopying, recording or otherwise without the prior written permission of the publisher Permissions may be sought directly from Elsevier’s Science & Technology Rights Department in Oxford, UK: phone (+44) (0) 1865 843830; fax (+44) (0) 1865 853333; email; [email protected]. Alternatively visit the Science and Technology Books website at www.elsevierdirect.com/rights for further information Notice No responsibility is assumed by the publisher for any injury and/or damage to persons or property as a matter of products liability, negligence or otherwise, or from any use or operation of any methods, products, instructions or ideas contained in the material herein. Because of rapid advances in the medical sciences, in particular, independent verifi cation of diagnoses and drug dosages should be made Library of Congress Cataloging-in-Publication Data A catalog record for this book is available from the Library of Congress British Library Cataloguing in Publication Data A catalogue record for this book is available from the British Library ISBN: 978-0-12-374349-7 For information on all Academic Press publications visit our website at www.elsevierdirect.com Typeset by Thomson Digital, Noida, India Printed and bound in the United States of America 09 10 11 12 13 9 8 7 6 5 4 3 2 1 Preface The potato (Solanum tuberosum L.) which is grown in over 100 countries throughout the world is one of the staples of the human diet. -
EC86-112 Origins, Adaptation, and Types of Corn W
University of Nebraska - Lincoln DigitalCommons@University of Nebraska - Lincoln Historical Materials from University of Nebraska- Extension Lincoln Extension 1986 EC86-112 Origins, Adaptation, and Types of Corn W. L. Brown M. S. Zuber L. L. Darrah Follow this and additional works at: http://digitalcommons.unl.edu/extensionhist Brown, W. L.; Zuber, M. S.; and Darrah, L. L., "EC86-112 Origins, Adaptation, and Types of Corn" (1986). Historical Materials from University of Nebraska-Lincoln Extension. 4596. http://digitalcommons.unl.edu/extensionhist/4596 This Article is brought to you for free and open access by the Extension at DigitalCommons@University of Nebraska - Lincoln. It has been accepted for inclusion in Historical Materials from University of Nebraska-Lincoln Extension by an authorized administrator of DigitalCommons@University of Nebraska - Lincoln. Nebraska Cooperative Extension Service EC86-11 2 Origins, Adaptations, and Types of Corn I , '... .._.. '""'' io f"rth"'"" of Coop""'" E><•ooioo wmk, Ao" of M•v 8 >Od J""' 30, 191 4 , '" ""'""''" whh<h• ;~··, ....... U.S. Department of Agriculture. Leo E. Lucas, Director of Cooperativ e Extension Service, University of Nebraska, ; . · ~ Institute of Agriculture and Natural Resources. •• ••~ •.o " The Cooperativ e Ex tension Service provi.des inf ormation and educational programs to all people w ithout regard to race, color, national ortg1n . sex or h andic ap. Origins, Adaptation, and Types of Corn* W .L. Brown, Pioneer Hi-Bred International, Inc., lA; M .S. Zuber, University of Missouri; L.L. Darrah, USDA-ARS, University of Missouri; and D.V. Glover, Purdue University ORIGIN OF CORN if less attention were given to determining corn's ori Corn (Zea mays L. -
Introduction to Corn
Introduction to Corn Why is corn such a valuable crop? How does corn grow, pollinate, and produce kernels? What farming techniques are important to increase corn yield? Corn is a grass, native to the Americas. Evidence in central Mexico suggests corn was used there about 9,000 years ago. Various Native American tribes shared their knowledge of corn, known as maize, with early European settlers, saving many from starvation. Early American colonists ground dried corn as meal for flour to use in porridge, cake, and bread. Sweet corn, served as “corn on the cob,” was not developed until the 1700s. Along with wheat and rice, corn is one of the world’s major grain crops. It is the largest grain crop grown in the United States. About 9 percent of all the corn is used to produce food for humans: corn meal, cooking oils, margarine, corn syrups, and sweeteners (fructose). About 64 percent of all corn is used for livestock feed. Corn is harvested for either grain or silage, with most of the grain going to dairies, animal feeding operations, and poultry operations. Corncobs have been used in the manufacturing of nylon fibers as well as being a source for producing biodegradable plastics. Ethanol, made from corn, is a renewable fuel used in today’s cars. Corn is pollinated by wind and is typically planted in 30-inch rows. A single seed, or kernel, of corn may produce a plant that yields more than 600 kernels of corn per ear. Approximately 22,000 to 35,000 individual plants may be grown on an acre of land. -
United States Patent Office Patented Dec
3,414,515 United States Patent Office Patented Dec. 3, 1968 1. 2 increase the water-solubility of starches and amylose by 3,414,515 chain-shortening procedures such as dextrinization or by COLOR IMPARTING COMPLEXES OF STARCH partial depolymerization with enzymes prior to complex ETHERS FOR SWIMMING POOLS Charles L. Mehitretter and William B. Roth, Peoria, Ill., ing with iodine were of no benefit since the therewith ob assignors to the United States of America as represented 5 tained more soluble iodine complexes were distinctly pur by the Secretary of Agriculture ple and esthetically unusable in swimming pools. No Drawing. Filed Oct. 5, 1964, Ser. No. 401,744 The primary object of our invention is the provision 1 Claim. (C. 210-62) of much more highly soluble ether derivatives of rela tively inexpensive polysaccharides, e.g., starches and amy lose that will complex with iodine to provide purely blue, ABSTRACT OF THE DESCLOSURE 10 potentially antiseptic products that, even when greatly Swimming pools can be disinfected with nonirritant diluted, provide a strong unequivocally blue color that iodine instead of chlorine and at the same time be given is free of any suggestion of purple or brown components a stable, highly attractive green to blue coloration that and that also is sufficiently intense even on high dilution maskes the expected yellow tint of the uncomplexed ex to completely mask the distinctly yellowish tint from any cess iodine by using in lieu of chlorine the deeply blue disinfecting excess of free iodine remaining after the poly iodine complex of a hydroxyalkyl ether of amylose and saccharide ether is added to an already iodinated swim of amylose-containing starches having a D.S. -
Żywność Technologia Jakość
lЩА& POLSKIE TOWARZYSTWO TECHNOLOGÓW ŻYWNOŚCI WYDAWCA ODDZIAŁ MAŁOPOLSKI ŻYWNOŚĆ TECHNOLOGIA JAKOŚĆ Suplement Nr 4(17) Kraków 1998 Rok 5 Materiały VIII International Starch Convention Kraków, 16-19 czerwca 1998 r. Komitet Naukowy: A.A.C.M. Beenackers (Groningen) Hidetsuku Fuwa (Fukuyama) Janos Hollo (Budapest) Jay-lin Jane (Ames, Iowa) Wolfgang Kempf (Detmold) Josef Korolczuk (Vileneuve d’Ascq) Chang-yi Lii (Taipei) Friedrich Meuser (Berlin) Werner Praznik (Vienna/Tulln) Harald Roper (Vilvoorde) Antoni Rutkowski (Warsaw) Piotr Tomasik (Cracow) Komitet Organizacyjny: Piotr Tomasik (head) Bohdan Achrem-Achremowicz Mieczysław Pałasiński Józef Gładkowski Tadeusz Sikora Małgorzata Bączkowicz (secretary) Organizatorzy Konferencii: Akademia Rolnicza w Krakowie Polskie Towarzystwo Technologów Żywności - Oddział Małopolski Polski Przemysł Ziemniaczany „POLZIEM” Sp. z o.o. w Poznaniu Komitet Technologii i Chemii Żywności PAN Redakcja naukowa materiałów: prof, dr hab. Piotr Tomasik Wydanie dofinansowane przez Komitet Badań Naukowych POLSKIE TOWARZYSTWO TECHNOLOGÓW ŻYWNOŚCI WYDAWCA ODDZIAŁ MAŁOPOLSKI w w ZYWNOSC TECHNOLOGIA JAKOSC Suplement Nr 4(17) Kraków 1998 Rok 5 REDAKCJA: Redaktor naczelny: prof, dr hab. Tadeusz Sikora; tel. 012/633-08-21 w. 21 Sekretarz redakcji: dr inż. Anna Bala-Piasek; tel. 012/ 411-91-44 w. 293; e-mail: [email protected] Redaktorzy: prof, dr hab. Bohdan Achremowicz, prof, dr hab. Mieczysław Pałasiński, dr inż. Jerzy Pałasiński, dr Teresa Woźniakiewicz Stali współpracownicy: prof, dr hab. Jacek Kijowski (Poznań), dr inż. Danuta Kołożyn-Krajewska (Warszawa), doc. dr hab. Maria Soral-Śmietana (Olsztyn) RADA PROGRAMOWA: prof, dr Antoni Rutkowski (przewodniczący), dr hab. Kazimierz Dąbrowski (sekretarz), prof, dr hab. Zbigniew Duda, prof, dr hab. Józef Fornal, prof, dr hab. Roman Grzybowski, prof, dr hab.