Regulation of Katanin Activity on Microtubules
Total Page:16
File Type:pdf, Size:1020Kb
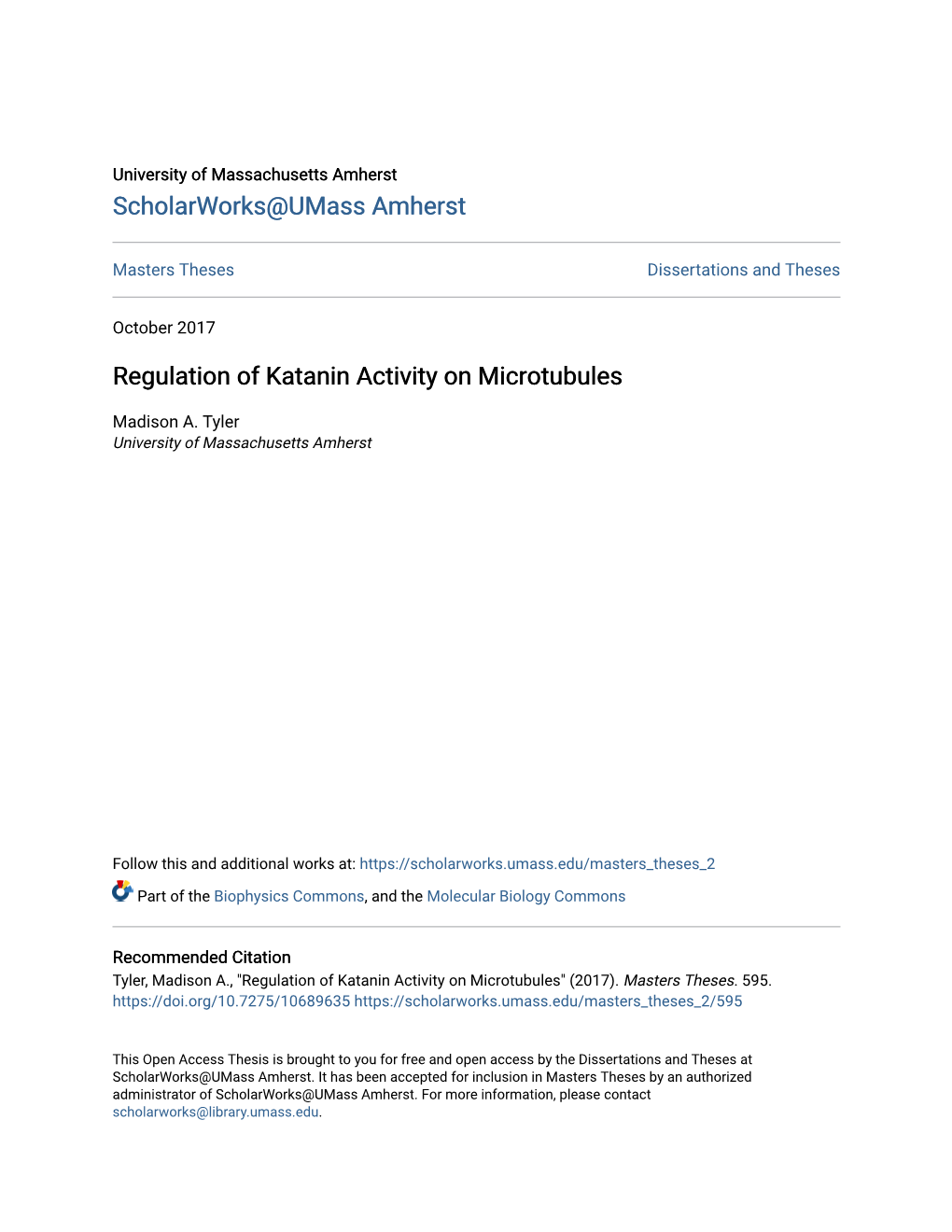
Load more
Recommended publications
-
Muscarinic Acetylcholine Type 1 Receptor Activity Constrains Neurite Outgrowth by Inhibiting Microtubule Polymerization and Mito
fnins-12-00402 June 26, 2018 Time: 12:46 # 1 ORIGINAL RESEARCH published: 26 June 2018 doi: 10.3389/fnins.2018.00402 Muscarinic Acetylcholine Type 1 Receptor Activity Constrains Neurite Outgrowth by Inhibiting Microtubule Polymerization and Mitochondrial Trafficking in Adult Sensory Neurons Mohammad G. Sabbir1*, Nigel A. Calcutt2 and Paul Fernyhough1,3 1 Division of Neurodegenerative Disorders, St. Boniface Hospital Research Centre, Winnipeg, MB, Canada, 2 Department of Pathology, University of California, San Diego, San Diego, CA, United States, 3 Department of Pharmacology and Therapeutics, University of Manitoba, Winnipeg, MB, Canada The muscarinic acetylcholine type 1 receptor (M1R) is a metabotropic G protein-coupled Edited by: receptor. Knockout of M1R or exposure to selective or specific receptor antagonists Roberto Di Maio, elevates neurite outgrowth in adult sensory neurons and is therapeutic in diverse University of Pittsburgh, United States models of peripheral neuropathy. We tested the hypothesis that endogenous M1R Reviewed by: activation constrained neurite outgrowth via a negative impact on the cytoskeleton Roland Brandt, University of Osnabrück, Germany and subsequent mitochondrial trafficking. We overexpressed M1R in primary cultures Rick Dobrowsky, of adult rat sensory neurons and cell lines and studied the physiological and The University of Kansas, United States molecular consequences related to regulation of cytoskeletal/mitochondrial dynamics *Correspondence: and neurite outgrowth. In adult primary neurons, overexpression of M1R caused Mohammad G. Sabbir disruption of the tubulin, but not actin, cytoskeleton and significantly reduced neurite [email protected] outgrowth. Over-expression of a M1R-DREADD mutant comparatively increased neurite Specialty section: outgrowth suggesting that acetylcholine released from cultured neurons interacts This article was submitted to with M1R to suppress neurite outgrowth. -
Phosphorylation of the Microtubule-Severing AAA+ Enzyme Katanin Regulates C
Phosphorylation of the microtubule-severing AAA+ enzyme Katanin regulates C. elegans embryo development Nicolas Joly, Eva Beaumale, Lucie van Hove, Lisa Martino, Lionel Pintard To cite this version: Nicolas Joly, Eva Beaumale, Lucie van Hove, Lisa Martino, Lionel Pintard. Phosphorylation of the microtubule-severing AAA+ enzyme Katanin regulates C. elegans embryo development. Journal of Cell Biology, Rockefeller University Press, 2020, 219 (6), 10.1083/jcb.201912037. hal-03011242 HAL Id: hal-03011242 https://hal.archives-ouvertes.fr/hal-03011242 Submitted on 18 Nov 2020 HAL is a multi-disciplinary open access L’archive ouverte pluridisciplinaire HAL, est archive for the deposit and dissemination of sci- destinée au dépôt et à la diffusion de documents entific research documents, whether they are pub- scientifiques de niveau recherche, publiés ou non, lished or not. The documents may come from émanant des établissements d’enseignement et de teaching and research institutions in France or recherche français ou étrangers, des laboratoires abroad, or from public or private research centers. publics ou privés. ARTICLE Phosphorylation of the microtubule-severing AAA+ enzyme Katanin regulates C. elegans embryo development Nicolas Joly, Eva Beaumale, Lucie Van Hove, Lisa Martino, and Lionel Pintard The evolutionarily conserved microtubule (MT)-severing AAA-ATPase enzyme Katanin is emerging as a critical regulator of MT dynamics. In Caenorhabditis elegans, Katanin MT-severing activity is essential for meiotic spindle assembly but is toxic for the Downloaded from https://rupress.org/jcb/article-pdf/219/6/e201912037/1044138/jcb_201912037.pdf by guest on 20 July 2020 mitotic spindle. Here we analyzed Katanin dynamics in C. elegans and deciphered the role of Katanin phosphorylation in the regulation of its activity and stability. -
Deciphering the Role of a Microtubule Severing Protein and a Protein Kinase in Cell Cycle and Ciliogenesis in Chlamydomonas Reinhardtii
DECIPHERING THE ROLE OF A MICROTUBULE SEVERING PROTEIN AND A PROTEIN KINASE IN CELL CYCLE AND CILIOGENESIS IN CHLAMYDOMONAS REINHARDTII by M. Qasim Rasi B.Sc., Simon Fraser University, 2004 THESIS SUBMITTED IN PARTIAL FULFILLMENT OF THE REQUIREMENTS FOR THE DEGREE OF DOCTOR OF PHILOSOPHY In the Department of Molecular Biology and Biochemistry ©M. Qasim Rasi 2009 SIMON FRASER UNIVERSITY Summer 2009 All rights reserved. This work may not be reproduced in whole or in part, by photocopy or other means, without permission of the author APPROVAL Name: M. Qasim Rasi Degree: Doctor of Philosophy Title of Thesis: Deciphering the role of a microtubule severing protein and a protein kinase in cell cycle and ciliogenesis in Chlamydomonas reinhardtii Examining Committee: Chair: Dr. C. Krieger Professor of Kinesiology _______________________________________________ Dr. Lynne Quarmby Senior Supervisor; Professor of Molecular Biology and Biochemistry _______________________________________________ Dr. Michel Leroux Supervisor; Associate Professor of Molecular Biology and Biochemistry _______________________________________________ Dr. Nick Harden Supervisor; Associate Professor of Molecular Biology and Biochemistry _______________________________________________ Dr. Christopher Beh Internal Examiner; Associate Professor of Molecular Biology and Biochemistry _______________________________________________ Dr. Stephen M. King External Examiner; Professor of Biochemistry, Associate Director, Graduate Program in Molecular Biology and Biochemistry, University of Connecticut Health Center Date Defended/Approved: June-5-2009 ii Declaration of Partial Copyright Licence The author, whose copyright is declared on the title page of this work, has granted to Simon Fraser University the right to lend this thesis, project or extended essay to users of the Simon Fraser University Library, and to make partial or single copies only for such users or in response to a request from the library of any other university, or other educational institution, on its own behalf or for one of its users. -
Evidence for Conformational Change-Induced Hydrolysis of Β-Tubulin-GTP
bioRxiv preprint doi: https://doi.org/10.1101/2020.09.08.288019; this version posted September 10, 2020. The copyright holder for this preprint (which was not certified by peer review) is the author/funder. All rights reserved. No reuse allowed without permission. Evidence for conformational change-induced hydrolysis of β-tubulin-GTP Mohammadjavad Paydar1 and Benjamin H. Kwok1† 1 Institute for Research in Immunology and Cancer (IRIC), Département de médecine, Université de Montréal, P.O. Box 6128, Station Centre-Ville, Montréal, QC H3C 3J7, Canada. † Corresponding author: B. H. Kwok: [email protected] Keywords: Kinesin, Kif2A, MCAK, motor protein, microtubules, tubulin, GTP hydrolysis Abbreviations NM: Neck+Motor MD: Motor domain MT: Microtubule Running title: Tubulin GTP hydrolysis 1 bioRxiv preprint doi: https://doi.org/10.1101/2020.09.08.288019; this version posted September 10, 2020. The copyright holder for this preprint (which was not certified by peer review) is the author/funder. All rights reserved. No reuse allowed without permission. ABSTRACT Microtubules, protein polymers of α/β-tubulin dimers, form the structural framework for many essential cellular processes including cell shape formation, intracellular transport, and segregation of chromosomes during cell division. It is known that tubulin-GTP hydrolysis is closely associated with microtubule polymerization dynamics. However, the precise roles of GTP hydrolysis in tubulin polymerization and microtubule depolymerization, and how it is initiated are still not clearly defined. We report here that tubulin-GTP hydrolysis can be triggered by conformational change induced by the depolymerizing kinesin-13 proteins or by the stabilizing chemical agent paclitaxel. We provide biochemical evidence that conformational change precedes tubulin-GTP hydrolysis, confirming this process is mechanically driven and structurally directional. -
NIH Public Access Author Manuscript Future Neurol
NIH Public Access Author Manuscript Future Neurol. Author manuscript; available in PMC 2013 January 08. Published in final edited form as: Future Neurol. 2012 November 1; 7(6): 749–771. doi:10.2217/FNL.12.68. Opening Pandora’s jar: a primer on the putative roles of CRMP2 in a panoply of neurodegenerative, sensory and motor neuron, $watermark-textand $watermark-text central $watermark-text disorders Rajesh Khanna1,2,3,4,*, Sarah M Wilson1,‡, Joel M Brittain1,‡, Jill Weimer5, Rukhsana Sultana6, Allan Butterfield6, and Kenneth Hensley7 1Program in Medical Neurosciences, Paul & Carole Stark Neurosciences Research Institute Indianapolis, IN 46202, USA 2Departments of Pharmacology & Toxicology, Indianapolis, IN 46202, USA 3Biochemistry & Molecular Biology, Indiana University School of Medicine, Indianapolis, IN 46202, USA 4Sophia Therapeutics LLC, Indianapolis, IN 46202, USA 5Sanford Children’s Health Research Center, Sanford Research & Department of Pediatrics, Sanford School of Medicine of the University of South Dakota, Sioux Falls, SD 57104, USA 6Department of Chemistry, Center of Membrane Sciences, & Sanders-Brown Center on Aging, University of Kentucky, Lexington, KY 40506, USA 7Department of Pathology & Department of Neurosciences, University of Toledo Medical Center, Toledo, OH 43614, USA Abstract CRMP2, also known as DPYSL2/DRP2, Unc-33, Ulip or TUC2, is a cytosolic phosphoprotein that mediates axon/dendrite specification and axonal growth. Mapping the CRMP2 interactome has revealed previously unappreciated functions subserved by this -
1/05661 1 Al
(12) INTERNATIONAL APPLICATION PUBLISHED UNDER THE PATENT COOPERATION TREATY (PCT) (19) World Intellectual Property Organization International Bureau (10) International Publication Number (43) International Publication Date _ . ... - 12 May 2011 (12.05.2011) W 2 11/05661 1 Al (51) International Patent Classification: (81) Designated States (unless otherwise indicated, for every C12Q 1/00 (2006.0 1) C12Q 1/48 (2006.0 1) kind of national protection available): AE, AG, AL, AM, C12Q 1/42 (2006.01) AO, AT, AU, AZ, BA, BB, BG, BH, BR, BW, BY, BZ, CA, CH, CL, CN, CO, CR, CU, CZ, DE, DK, DM, DO, (21) Number: International Application DZ, EC, EE, EG, ES, FI, GB, GD, GE, GH, GM, GT, PCT/US20 10/054171 HN, HR, HU, ID, IL, IN, IS, JP, KE, KG, KM, KN, KP, (22) International Filing Date: KR, KZ, LA, LC, LK, LR, LS, LT, LU, LY, MA, MD, 26 October 2010 (26.10.2010) ME, MG, MK, MN, MW, MX, MY, MZ, NA, NG, NI, NO, NZ, OM, PE, PG, PH, PL, PT, RO, RS, RU, SC, SD, (25) Filing Language: English SE, SG, SK, SL, SM, ST, SV, SY, TH, TJ, TM, TN, TR, (26) Publication Language: English TT, TZ, UA, UG, US, UZ, VC, VN, ZA, ZM, ZW. (30) Priority Data: (84) Designated States (unless otherwise indicated, for every 61/255,068 26 October 2009 (26.10.2009) US kind of regional protection available): ARIPO (BW, GH, GM, KE, LR, LS, MW, MZ, NA, SD, SL, SZ, TZ, UG, (71) Applicant (for all designated States except US): ZM, ZW), Eurasian (AM, AZ, BY, KG, KZ, MD, RU, TJ, MYREXIS, INC. -
The Tumor Suppressor LZTS2 Functions Through the Cellular Samurai Katanin
Cent. Eur. J. Biol. • 4(1) • 2009 • 1–10 DOI: 10.2478/s11535-008-0063-0 Central European Journal of Biology The tumor suppressor LZTS2 functions through the cellular samurai Katanin Mini-Review Yoshiro Maru* Department of Pharmacology, Tokyo Womens Medical University, 162-8666 Tokyo, Japan Received 10 September 2008; Accepted 16 December 2008 Abstract: The leucine zipper putative tumor suppressor (LZTS) 2 is frequently and specifically found in LOH (loss of heterozygosity) analysis in cancer. Different from other LZTS family members, it regulates the microtubule-severing protein Katanin by binding the p80 regulatory subunit of Katanin and inhibiting its interaction with microtubules. At specific phases of the cell cycle, LZTS2 suppresses cell migration and establishes proper central spindle assembly for cytokinesis. Importantly, those biological effects are mediated by the inhibitory activity of LZTS2 on Katanin. LZTS2 binding to Katanin also plays a role in Katanin transport to the midbody to control proper abscis- sion. Therapeutic applications of the interaction between LZTS2 and Katanin in tumor cells are a potential area for future research. Keywords: LAPSER1 • LZTS2 • Katanin • β-catenin • Microtubule • Cancer © Versita Warsaw and Springer-Verlag Berlin Heidelberg. 1. Introduction β-tubulin cause resistance to taxanes [2]. Farnesyltransferase inhibitors, which were initially found to inhibit the Ras oncoprotein, can enhance the binding Pharmaceutical discovery has been aided by elucidation between β-tubulin and taxanes to reverse resistance to of mechanisms by which tumor suppressors inhibit taxanes [3]. The genomic region that involves Aurora-A cancer progression. For example, the discovery of is commonly amplified in human tumors with taxane taxanes as microtubule-stabilizing drugs has shown resistance. -
The Importance of Lattice Defects in Katanin-Mediated Microtubule Severing in Vitro
2916 Biophysical Journal Volume 82 June 2002 2916–2927 The Importance of Lattice Defects in Katanin-Mediated Microtubule Severing in Vitro Liza J. Davis,* David J. Odde,† Steven M. Block,‡ and Steven P. Gross§ Departments of *Chemical Engineering and Materials Science and †Biomedical Engineering, University of Minnesota, Minneapolis, Minnesota 55455; ‡Department of Biological Sciences, Stanford University, Stanford, California 94305; and §Department of Developmental and Cell Biology, University of California-Irvine, Irvine, California 92697 USA ABSTRACT The microtubule-severing enzyme katanin uses ATP hydrolysis to disrupt noncovalent bonds between tubulin dimers within the microtubule lattice. Although its microtubule severing activity is likely important for fundamental processes including mitosis and axonal outgrowth, its mechanism of action is poorly understood. To better understand this activity, an in vitro assay was developed to enable the real-time observation of katanin-mediated severing of individual, mechanically unconstrained microtubules. To interpret the experimental observations, a number of theoretical models were developed and compared quantitatively to the experimental data via Monte Carlo simulation. Models that assumed that katanin acts on a uniform microtubule lattice were incompatible with the in vitro data, whereas a model that assumed that katanin acts preferentially on spatially infrequent microtubule lattice defects was found to correctly predict the experimentally observed breaking rates, number and spatial frequency of severing events, final levels of severing, and sensitivity to katanin concen- tration over the range 6–300 nM. As a result of our analysis, we propose that defects in the microtubule lattice, which are known to exist but previously not known to have any biological function, serve as sites for katanin activity. -
Microtubule Motors
Microtubule Forces Kevin Slep Microtubules are a Dynamic Scaffold Microtubules in red, XMA215 family MT polymerase protein in green Some Microtubule Functions Cell Structure Polarized Motor Track (kinesins and dynein) Cilia structure (motile and sensory) Mitotic and meiotic spindle structure Cell polarity Coordinate cell motility with the F-actin network Architecture of Tubulin and the Microtubule α/β-Tubulin: The Microtubule Building Block Tubulin is a heterodimer composed of α and β tubulin α and β tubulin are each approximately • 55 kD and are structurally very similar to •each other. •Each tubulin binds GTP: The α GTP is non- exchangeable and the dimer is very stable, Kd = 10-10; the β GTP is exchangeable in the dimer The Microtubule Architecture Tubulin binds head-to-tail along + protofilaments, forming LONGITUDINAL interactions. Longitudinal interactions complete the active site for GTP hydrolysis 13 protofilaments form a hollow tube-the microtubule: 25 nm OD, 14 nm ID (protofilaments interact via LATERAL interactions) The MT is a left-handed helix with a seam, it rises 1.5 heterodimers per turn (α and β form lateral interactions) MTs are polar-they have a plus end and a minus end - The γTubulin Ring Complex (γTuRC) forms a lockwasher to nucleate MTs Axial view Side View γTuRC positions nucleated 13 γTubulins in a ring γTuRC attachment microtubule The Centrosome is a Microtubule Organizing Center (MTOC) rich in γTuRC MTOC’s control where microtubules are formed Centrosomes contain peri-centrosomal material (PCM) surrounding a pair of centrioles γTuRC nucleation complexes are localized to the PCM Centrioles within centrosomes become basal bodies, which are nucleation centers for cilia (motile and primary) and flagella Centrosomes duplicate once per cell cycle Mother centriole nucleates growth of a daughter centriole with an orthogonal orientation Microtubule Polarity and Dynamics Polarized Microtubule Organization in Vivo Centrosome + + + + + + + + + Interphase Mitosis Microtubules are Dynamic Fish melanophore injected with Cy3-tubulin Vorobjev, I.A. -
The Mechanisms of Microtubule Catastrophe and Rescue: Implications from Analysis of a Dimer-Scale Computational Model
M BoC | ARTICLE The mechanisms of microtubule catastrophe and rescue: implications from analysis of a dimer-scale computational model Gennady Margolina,b,*,†, Ivan V. Gregorettib,c,*,†, Trevor M. Cickovskid,‡, Chunlei Lia,b, Wei Shia,b,§, Mark S. Albera,b,e, and Holly V. Goodsonb,c aDepartment of Applied and Computational Mathematics and Statistics, bInterdisciplinary Center for the Study of Biocomplexity, cDepartment of Chemistry and Biochemistry, and dDepartment of Computer Science and Engineering, University of Notre Dame, Notre Dame, IN 46556; eDepartment of Medicine, Indiana University School of Medicine, Indianapolis, IN 40202 ABSTRACT Microtubule (MT) dynamic instability is fundamental to many cell functions, but Monitoring Editor its mechanism remains poorly understood, in part because it is difficult to gain information Alexander Mogilner about the dimer-scale events at the MT tip. To address this issue, we used a dimer-scale com- University of California, Davis putational model of MT assembly that is consistent with tubulin structure and biochemistry, Received: Aug 12, 2011 displays dynamic instability, and covers experimentally relevant spans of time. It allows us to Revised: Nov 30, 2011 correlate macroscopic behaviors (dynamic instability parameters) with microscopic structures Accepted: Dec 13, 2011 (tip conformations) and examine protofilament structure as the tip spontaneously progresses through both catastrophe and rescue. The model’s behavior suggests that several commonly held assumptions about MT dynamics should be reconsidered. Moreover, it predicts that short, interprotofilament “cracks” (laterally unbonded regions between protofilaments) exist even at the tips of growing MTs and that rapid fluctuations in the depths of these cracks influ- ence both catastrophe and rescue. -
EFFECTS of BLOOD FEEDING on the TRANSCRIPTOME of the MALPIGHIAN TUBULES in the ASIAN TIGER MOSQUITO AEDES ALBOPICTUS Thesis
EFFECTS OF BLOOD FEEDING ON THE TRANSCRIPTOME OF THE MALPIGHIAN TUBULES IN THE ASIAN TIGER MOSQUITO AEDES ALBOPICTUS Thesis Presented in Partial Fulfillment of the Requirements for the Degree Master in Science in the Graduate School of The Ohio State University By Carlos J. Esquivel Palma, B.S. Graduate Program in Entomology The Ohio State University 2015 Master’s Examination Committee: Dr. Peter M. Piermarini, Advisor Dr. David L. Denlinger Dr. Andrew P. Michel Copyright by Carlos J. Esquivel Palma 2015 Abstract Mosquitoes are one of the major threats to human health worldwide. They are vectors of protozoans, arboviruses, and filarial nematodes that cause diseases in humans and animals. The Asian tiger mosquito Aedes albopictus is a vector of medically important arboviruses such as dengue fever, chikungunya fever, yellow fever, eastern equine encephalitis, La Crosse encephalitis, and West Nile fever. Control of these diseases often involves control of the mosquito vectors with chemical insecticides. However, the use of a limited number of chemicals with similar modes of actions has led to resistance in several mosquito species. The development of new insecticides with novel modes of action is considered a promising strategy to overcome resistance. The Piermarini lab has recently shown that the renal (Malpighian) tubules of mosquitoes are promising physiological targets to disrupt for killing mosquitoes via a novel mode of action. The Malpighian tubules play a critical role in the acute processing of blood meals, by mediating the rapid excretion of water and ions derived from the ingested blood. However, the physiological roles of Malpighian tubules during the chronic processing of blood meals after the initial diuresis is complete (~1-2 h after feeding) are not known. -
Phosphatidylinositol 4,5-Bisphosphate Modifies Tubulin
The Journal of Neuroscience, March 1, 2002, 22(5):1668–1678 Phosphatidylinositol 4,5-Bisphosphate Modifies Tubulin  Participation in Phospholipase C 1 Signaling Juliana S. Popova,1 Arin K. Greene,1 Jia Wang,1 and Mark M. Rasenick1,2 Departments of 1Physiology and Biophysics and 2Psychiatry, University of Illinois at Chicago, College of Medicine, Chicago, Illinois 60612-7342 Tubulin forms the microtubule and regulates certain G-protein- the plasma membrane was demonstrated with confocal laser mediated signaling pathways. Both functions rely on the GTP- immunofluorescence microscopy. Although tubulin bound to ␣ ␣  binding properties of tubulin. Signal transduction through G q- both G q and PLC 1 , PIP2 facilitated the interaction between    ␣ regulated phospholipase C 1 (PLC 1 ) is activated by tubulin tubulin and PLC 1 but not that between tubulin and G q. ␣ ␣  through a direct transfer of GTP from tubulin to G q. However, However, PIP2 did augment formation of tubulin–G q–PLC 1   at high tubulin concentrations, inhibition of PLC 1 is observed. complexes. Subsequent to potentiating PLC 1 activation, sus-  This report demonstrates that tubulin inhibits PLC 1 by binding tained agonist-independent membrane binding of tubulin   ␣ the PLC 1 substrate phosphatidylinositol 4,5-bisphosphate at PIP2- and PLC 1-rich sites appeared to inhibit G q coupling  (PIP2 ). Tubulin binding of PIP2 was specific, because PIP2 but to PLC 1. Furthermore, colchicine increased membrane-  not phosphatidylinositol 3,4,5-trisphosphate, phosphatidylino- associated tubulin and also inhibited PLC 1 activity in SK- sitol 3-phosphate, phosphatidylinositol, phosphatidylcholine, N-SH cells. Thus, tubulin, depending on local membrane con- phosphatidylethanolamine, or inositol 1,4,5-trisphosphate in- centration, may serve as a positive or negative regulator of hibited microtubule assembly.