Mineralogy and Geology of Asteroid (4) Vesta from Dawn Framing Camera
Total Page:16
File Type:pdf, Size:1020Kb
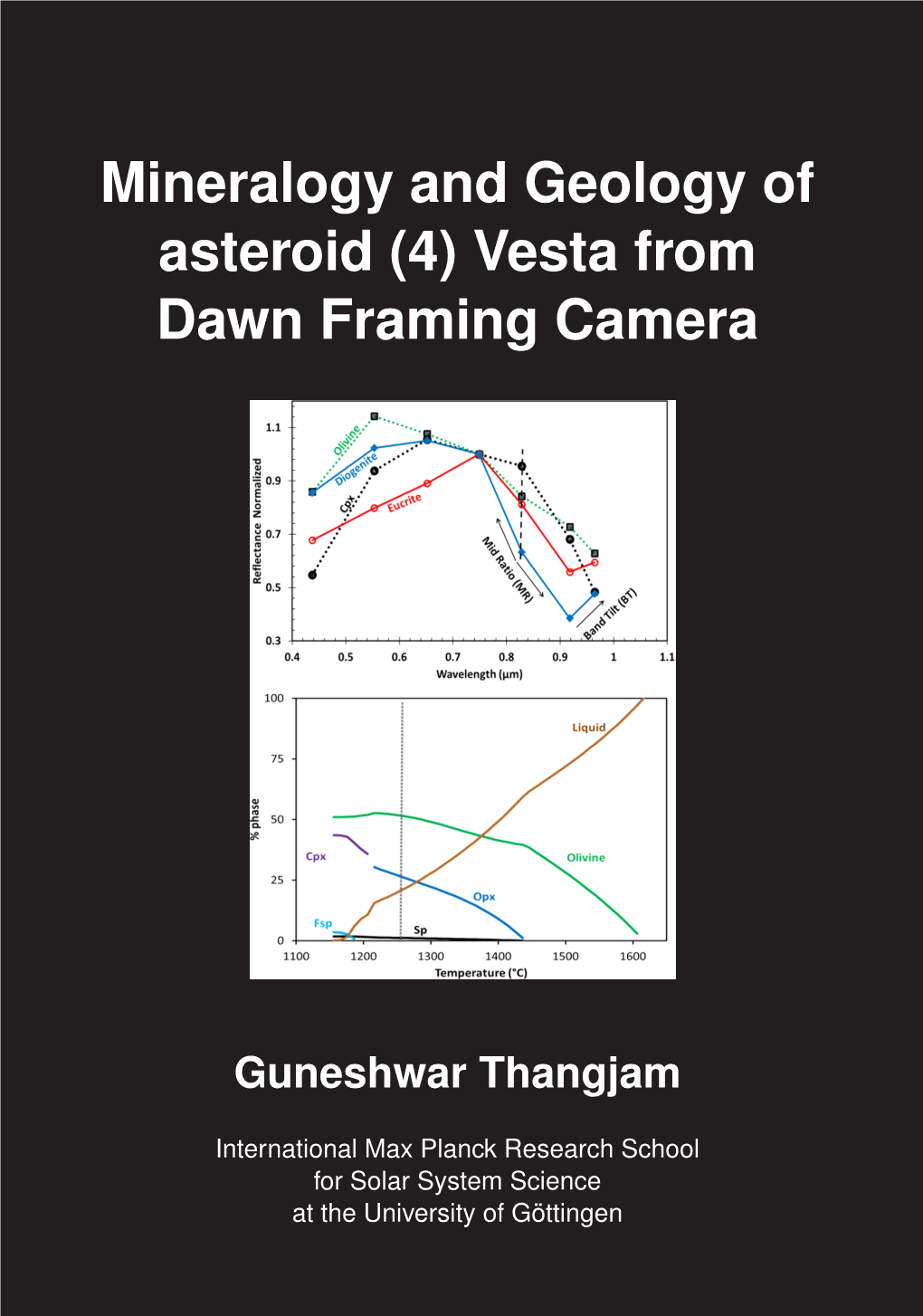
Load more
Recommended publications
-
Research Article Subsurface Thermal Modeling of Oxia Planum, Landing Site of Exomars 2022
Hindawi Advances in Astronomy Volume 2021, Article ID 9924571, 10 pages https://doi.org/10.1155/2021/9924571 Research Article Subsurface Thermal Modeling of Oxia Planum, Landing Site of ExoMars 2022 M. Formisano ,1 M. C. De Sanctis ,1 C. Federico,1 G. Magni,1 F. Altieri ,1 E. Ammannito ,2 S. De Angelis ,1 M. Ferrari ,1 and A. Frigeri 1 1INAF-IAPS, Via del Fosso del Cavaliere 100, Rome, Italy 2Italian Space Agency (ASI), Rome, Italy Correspondence should be addressed to M. Formisano; [email protected] Received 8 March 2021; Revised 18 May 2021; Accepted 25 June 2021; Published 2 September 2021 Academic Editor: Eriita Jones Copyright © 2021 M. Formisano et al. &is is an open access article distributed under the Creative Commons Attribution License, which permits unrestricted use, distribution, and reproduction in any medium, provided the original work is properly cited. Numerical simulations are required to thermophysically characterize Oxia Planum, the landing site of the mission ExoMars 2022. A drilling system is installed on the ExoMars rover, and it will be able to analyze down to 2 meters in the subsurface of Mars. &e spectrometer Ma_MISS (Mars Multispectral Imager for Subsurface, Coradini and Da Pieve, 2001) will investigate the lateral wall of the borehole generated by the drill, providing hyperspectral images. It is not fully clear if water ice can be found in the subsurface at Oxia Planum. However, Ma_MISS has the capability to characterize and map the presence of possible ices, in particular water ice. We performed simulations of the subsurface temperatures by varying the thermal inertia, and we quantified the effects of self-heating. -
Selection of Processing Tomato Genotypes Resistant to Two Spotted Spider Mite
Scientific communication VALADARES, RN; MELO, RA; SARINHO, IVF; OLIVEIRA, NS; ROCHA, FAT; SILVA, JW; MENEZES, D. 2018. Genetic diversity in accessions of melon belonging to momordica group. Horticultura Brasileira 36: 253258. DOI: http://dx.doi.org/10.1590/S0102-053620180218 Genetic diversity in accessions of melon belonging to momordica group Ricardo N Valadares1; Roberto A Melo1; Isabel VF Sarinho1; Natália S Oliveira2; Fernando AT Rocha1; José W Silva1; Dimas Menezes1 1Universidade Federal Rural de Pernambuco (UFRPE), Recife-PE, Brazil; [email protected] (corresponding author); [email protected]; [email protected]; [email protected]; [email protected]; dimasmenezes@ superig.com.b; 2Universidade Federal de Lavras (UFLA), Lavras-MG, Brazil; [email protected] ABSTRACT RESUMO The genetic divergence of melon genotypes belonging to Divergência genética em acessos de melão do grupo momordica group, collected in five Brazilian States, was estimated, momordica and the relative contribution of the morphological characters was A divergência genética de genótipos de melão do grupo determined for the genetic variability. The experimental design was momordica foi estimada, coletados em cinco estados brasileiros, randomized blocks, with four replicates. We evaluated 19 accessions e determinada a contribuição relativa dos caracteres morfológicos of melon, momordica group, two accessions of cantaloupensis group avaliados para a variabilidade genética. Foi adotado o delineamento and two commercial cultivars of inodorus group. These genotypes de blocos casualizados com quatro repetições. Nesse estudo, were characterized by 42 morphological descriptors. The data were foram utilizados 19 acessos de melão do grupo momordica, dois submitted to Tocher and UPGMA grouping methods using the genetic acessos do grupo cantaloupensis e duas cultivares comerciais do dissimilarity matrix, using Mahalanobis’ distance. -
Martian Crater Morphology
ANALYSIS OF THE DEPTH-DIAMETER RELATIONSHIP OF MARTIAN CRATERS A Capstone Experience Thesis Presented by Jared Howenstine Completion Date: May 2006 Approved By: Professor M. Darby Dyar, Astronomy Professor Christopher Condit, Geology Professor Judith Young, Astronomy Abstract Title: Analysis of the Depth-Diameter Relationship of Martian Craters Author: Jared Howenstine, Astronomy Approved By: Judith Young, Astronomy Approved By: M. Darby Dyar, Astronomy Approved By: Christopher Condit, Geology CE Type: Departmental Honors Project Using a gridded version of maritan topography with the computer program Gridview, this project studied the depth-diameter relationship of martian impact craters. The work encompasses 361 profiles of impacts with diameters larger than 15 kilometers and is a continuation of work that was started at the Lunar and Planetary Institute in Houston, Texas under the guidance of Dr. Walter S. Keifer. Using the most ‘pristine,’ or deepest craters in the data a depth-diameter relationship was determined: d = 0.610D 0.327 , where d is the depth of the crater and D is the diameter of the crater, both in kilometers. This relationship can then be used to estimate the theoretical depth of any impact radius, and therefore can be used to estimate the pristine shape of the crater. With a depth-diameter ratio for a particular crater, the measured depth can then be compared to this theoretical value and an estimate of the amount of material within the crater, or fill, can then be calculated. The data includes 140 named impact craters, 3 basins, and 218 other impacts. The named data encompasses all named impact structures of greater than 100 kilometers in diameter. -
The Eternal Fire of Vesta
2016 Ian McElroy All Rights Reserved THE ETERNAL FIRE OF VESTA Roman Cultural Identity and the Legitimacy of Augustus By Ian McElroy A thesis submitted to the Graduate School-New Brunswick Rutgers, The State University of New Jersey In partial fulfillment of the requirements For the degree of Master of Arts Graduate Program in Classics Written under the direction of Dr. Serena Connolly And approved by ___________________________________________ ___________________________________________ ___________________________________________ New Brunswick, New Jersey October 2016 ABSTRACT OF THE THESIS The Eternal Fire of Vesta: Roman Cultural Identity and the Legitimacy of Augustus By Ian McElroy Thesis Director: Dr. Serena Connolly Vesta and the Vestal Virgins represented the very core of Roman cultural identity, and Augustus positioned his public image beside them to augment his political legitimacy. Through analysis of material culture, historiography, and poetry that originated during the principate of Augustus, it becomes clear that each of these sources of evidence contributes to the public image projected by the leader whom Ronald Syme considered to be the first Roman emperor. The Ara Pacis Augustae and the Res Gestae Divi Augustae embody the legacy the Emperor wished to establish, and each of these cultural works contain significant references to the Vestal Virgins. The study of history Livy undertook also emphasized the pathetic plight of Rhea Silvia as she was compelled to become a Vestal. Livy and his contemporary Dionysius of Halicarnassus explored the foundation of the Vestal Order and each writer had his own explanation about how Numa founded it. The Roman poets Virgil, Horace, Ovid, and Tibullus incorporated Vesta and the Vestals into their work in a way that offers further proof of the way Augustus insinuated himself into the fabric of Roman cultural identity by associating his public image with these honored priestesses. -
Low Resolution
No. 102 June 2021 IAMGIAMG NewsletterNewsletter Official Newsletter of the International Association for Mathematical Geosciences Contents ith the Covid-19 pandemic Wfar from over, most Announcement of the 2021 IAmG AwArds ......................... 1 scientific meetings have been PresIdent’s forum .............................................................. 3 postponed or converted to a member news ...................................................................... 3 digital format. While there are nomInAtIons for IAmG AwArds ........................................... 3 certainly benefits to online meetings (I definitely don’t miss multiple memorAndum wIth codA AssocIAtIon ................................ 3 flights each way and jetlag) they tend reseArch center for solId eArth bIG dAtA to lose the personal interactions. It founded At the chInA unIversIty of GeoscIences ........... 4 is difficult for an online conference rememberInG dr. Peter fox - A tItAn In the eArth to replicate the conversations in the scIence InformAtIcs communIty ........................................ 4 hallways between sessions or during a meal that can bring a community Ieee GeoscIence And remote sensInG socIety (Grss) dIs- together and build new connections and tInGuIshed lecturer (dl) ............................................. 4 ideas. If you have any ideas or examples Professor noel cressIe nAmed A fellow of the of how the IAMG could work to bring the royAl socIety of new south Wales................................... 4 community together, please -
March 21–25, 2016
FORTY-SEVENTH LUNAR AND PLANETARY SCIENCE CONFERENCE PROGRAM OF TECHNICAL SESSIONS MARCH 21–25, 2016 The Woodlands Waterway Marriott Hotel and Convention Center The Woodlands, Texas INSTITUTIONAL SUPPORT Universities Space Research Association Lunar and Planetary Institute National Aeronautics and Space Administration CONFERENCE CO-CHAIRS Stephen Mackwell, Lunar and Planetary Institute Eileen Stansbery, NASA Johnson Space Center PROGRAM COMMITTEE CHAIRS David Draper, NASA Johnson Space Center Walter Kiefer, Lunar and Planetary Institute PROGRAM COMMITTEE P. Doug Archer, NASA Johnson Space Center Nicolas LeCorvec, Lunar and Planetary Institute Katherine Bermingham, University of Maryland Yo Matsubara, Smithsonian Institute Janice Bishop, SETI and NASA Ames Research Center Francis McCubbin, NASA Johnson Space Center Jeremy Boyce, University of California, Los Angeles Andrew Needham, Carnegie Institution of Washington Lisa Danielson, NASA Johnson Space Center Lan-Anh Nguyen, NASA Johnson Space Center Deepak Dhingra, University of Idaho Paul Niles, NASA Johnson Space Center Stephen Elardo, Carnegie Institution of Washington Dorothy Oehler, NASA Johnson Space Center Marc Fries, NASA Johnson Space Center D. Alex Patthoff, Jet Propulsion Laboratory Cyrena Goodrich, Lunar and Planetary Institute Elizabeth Rampe, Aerodyne Industries, Jacobs JETS at John Gruener, NASA Johnson Space Center NASA Johnson Space Center Justin Hagerty, U.S. Geological Survey Carol Raymond, Jet Propulsion Laboratory Lindsay Hays, Jet Propulsion Laboratory Paul Schenk, -
Space Resources Roundtable II : November 8-10, 2000, Golden, Colorado
SPACE RESOURCES RouNDTABLE II November 8-10, 2000 Colorado School of Mines Golden, Colorado LPI Contribution No. 1070 SPACE RESOURCES ROUNDTABLE II November 8-10, 2000 Golden, Colorado Sponsored by Colorado School of Mines Lunar and Planetary Institute National Aeronautics and Space Administration Steering Committee Joe Burris, WorldTradeNetwork.net David Criswell, University of Houston Michael B. Duke, Lunar and Planetary Institute Mike O'Neal, NASA Kennedy Space Center Sanders Rosenberg, InSpace Propulsion, Inc. Kevin Reed, Marconi, Inc. Jerry Sanders, NASA Johnson Space Center Frank Schowengerdt, Colorado School of Mines Bill Sharp, Colorado School of Mines LPI Contribution No. 1070 Compiled in 2000 by LUNAR AND PLANETARY INSTITUTE The Institute is operated by the Universities Space Research Association under Contract No. NASW-4574 with the National Aeronautics and Space Administration. Matenal in this volume may be copied wnhout restraint for library, abstract service, education. or personal research purposes; however, republication of any paper or portion thereof requ1res the wriuen permission of the authors as well as the appropnate acknowledgment of this publication. Abstracts in this volume may be cited as Author A B. (2000) Title of abstract. In Space Resources Roundtable II. p x.x . LPI Contnbuuon No. 1070. Lunar and Planetary Institute. Houston. This repon is distributed by ORDER DEPARTMENT Lunar and Planetary Institute 3600 Bay Area Boulevard Houston TX 77058-1113 Phone: 281-486-2172 Fax: 281-486-2186 E-mail: [email protected] Mail order requestors will be invoiced for the cost of shipping and handling. LPI Contribution No 1070 iii Preface This volume contains abstracts that have been accepted for presentation at the Space Resources Roundtable II, November 8-10, 2000. -
High Survivability of Micrometeorites on Mars: Sites with Enhanced Availability of Limiting Nutrients
RESEARCH ARTICLE High Survivability of Micrometeorites on Mars: Sites With 10.1029/2019JE006005 Enhanced Availability of Limiting Nutrients Key Points: Andrew G. Tomkins1 , Matthew J. Genge2,3 , Alastair W. Tait1,4 , Sarah L. Alkemade1, • Micrometeorites are predicted to be 1 1 5 far more abundant on Mars than on Andrew D. Langendam , Prudence P. Perry , and Siobhan A. Wilson Earth 1 2 • Micrometeorites are concentrated in School of Earth, Atmosphere and Environment, Melbourne, Victoria, Australia, Impact and Astromaterials Research the residue of aeolian sediment Centre, Department of Earth Science and Engineering, Imperial College London, London, UK, 3Department of removal, such as at bedrock cracks Mineralogy, The Natural History Museum, London, UK, 4Department of Biological and Environmental Sciences, and gravel accumulations University of Stirling, Scotland, UK, 5Department of Earth and Atmospheric Sciences, University of Alberta, Edmonton, • Micrometeorite accumulation sites are enriched in key nutrients for Alberta, Canada primitive microbes: reduced phosphorus, sulfur, and iron Abstract NASA's strategy in exploring Mars has been to follow the water, because water is essential for life, and it has been found that there are many locations where there was once liquid water on the surface. Now perhaps, to narrow down the search for life on a barren basalt‐dominated surface, there needs to be a Correspondence to: A. G. Tomkins, refocusing to a strategy of “follow the nutrients.” Here we model the entry of metallic micrometeoroids [email protected] through the Martian atmosphere, and investigate variations in micrometeorite abundance at an analogue site on the Nullarbor Plain in Australia, to determine where the common limiting nutrients available in Citation: these (e.g., P, S, Fe) become concentrated on the surface of Mars. -
Connection Between Micrometeorites and Wild 2 Particles: from Antarctic Snow to Cometary Ices
Meteoritics & Planetary Science 44, Nr 10, 1643–1661 (2009) Abstract available online at http://meteoritics.org Connection between micrometeorites and Wild 2 particles: From Antarctic snow to cometary ices E. DOBRIC√1,*, C. ENGRAND1, J. DUPRAT1, M. GOUNELLE2, H. LEROUX3, E. QUIRICO4, and J.-N. ROUZAUD5 1Centre de Spectrométrie Nucléaire et de Spectrométrie de Masse, CNRS-Univ. Paris Sud, 91405 Orsay Campus, France 2Laboratoire de Minéralogie et de Cosmochimie, Muséum National d’Histoire Naturelle, 57 rue Cuvier, CP52, 75005 Paris, France 3Laboratoire de Structure et Propriétés de l’Etat Solide, CNRS-Univ. des Sciences et Technologies de Lille, 59655 Villeneuve d’Ascq, France 4Laboratoire de Planétologie de Grenoble, Univ. J. Fourier CNRS-INSU 38041 Grenoble Cedex 09, France 5Laboratoire de Géologie, Ecole Normale Supérieure, CNRS, 75231 Paris Cedex 5, France *Corresponding author. E-mail: [email protected] (Received 17 March 2009; revision accepted 21 September 2009) Abstract–We discuss the relationship between large cosmic dust that represents the main source of extraterrestrial matter presently accreted by the Earth and samples from comet 81P/Wild 2 returned by the Stardust mission in January 2006. Prior examinations of the Stardust samples have shown that Wild 2 cometary dust particles contain a large diversity of components, formed at various heliocentric distances. These analyses suggest large-scale radial mixing mechanism(s) in the early solar nebula and the existence of a continuum between primitive asteroidal and cometary matter. The recent collection of CONCORDIA Antarctic micrometeorites recovered from ultra-clean snow close to Dome C provides the most unbiased collection of large cosmic dust available for analyses in the laboratory. -
MMM 265 May2013
MMM #265 Since December 1986 May 2013 – p 1 A US Defense Advanced Research Projects Agency satellite scavenging a defunct satellite’s valuable parts. DARPA has $180 M to test space debris scavenging technologies. The Canadians and Swiss are also working in this area. Feature Articles: 2 In Focus: National Space Society Perennial Identity Crisis - Peter Kokh, Member NSS Board of Advisors 3 Mars Aviation could Debut in the deep Hellas Basin - Peter Kokh 4 The "Inspiration Mars" Project - Peter Kokh 6 Making the Most of an "Inspiration Mars" Project - Peter Kokh 7 Refueling Stations Beyond Low Earth Orbit: The Stage is Set: Mining Asteroids - or Dormant Comets? - Pros & Cons - Peter Kokh “Comatose” Comets might be a better choice than Asteroids pp 7-8 For past articles, Visit http://www.moonsociety.org/publications/mmm_classics/ or /mmm_themes/ MMM #265 Since December 1986 May 2013 – p 2 About Moon Miners’ Manifesto - “The Moon - it’s not Earth, but it’s Earth’s!” • MMM’s VISION: “expanding the human economy through of-planet resources”; early heavy reliance on Lunar materials; early use of Mars system and asteroid resources; and permanent settlements supporting this economy. • MMM’s MISSION: to encourage “spin-up” entrepreneurial development of the novel technologies needed and promote the economic-environmental rationale of space and lunar settlement. • Moon Miners’ Manifesto CLASSICS: The non-time-sensitive articles and editorials of MMM’s first twenty years plus have been re-edited, reillustrated, and republished in 23 PDF format volumes, for free downloading from this location: http://www.MoonSociety.org/publications/mmm_classics/ • MMM THEME Issues: 14 collections of articles according to themes: ..../publications/mmm_themes/ • MMM Glossary: new terms, old terms/new meanings: www.moonsociety.org/publications/m3glossary.html • MMM retains its editorial independence and serves many groups, each with its own philosophy, agenda, and programs. -
Overview of the Spacecraft Design for the Psyche Mission Concept William Hart1, G
Overview of the Spacecraft Design for the Psyche Mission Concept William Hart1, G. Mark Linda T. Elkins-Tanton12, David J. Lawrence14 Peter Lord15, Zachary Brown2, Steven M. Collins3, James F. Bell III13 Applied Physics Pirkl16 Maria De Soria-Santacruz Arizona State University Laboratory Space Systems/Loral, LLC Pich4, Paul Fieseler5, Dan PO Box 871404 Johns Hopkins University 3825 Fabian Avenue Goebel6, Danielle Marsh7, Tempe, AZ 85287 11100 Johns Hopkins Road Palo Alto, CA 94303 David Y. Oh8, Steve Laurel, Maryland 20723 Snyder9, Noah Warner10 and Gregory Whiffen11 Jet Propulsion Laboratory California Institute of Technology 4800 Oak Grove Drive Pasadena, CA 91109 Abstract — In January 2017, Psyche and a second mission 1. INTRODUCTION concept were selected by NASA for flight as part of the 14th Discovery mission competition. Assigned for an initial launch Started in 1992, NASA’s Discovery program has date in 2023, the Psyche team was given direction shortly after demonstrated the science benefits that may be attained selection to research the possibility for earlier opportunities. Ultimately, the team was able to identify a launch opportunity through cost-capped, competitively awarded exploration in 2022 with a reduced flight time to its destination. This was missions beyond Earth orbit. In over twenty years since the accomplished in large part to crosscutting trades centered on launch of the first mission, NEAR Pathfinder, there have the electrical power subsystem. These trades were facilitated been a compelling list of successes, such as Mars through the Psyche mission's planned use of Solar Electric Pathfinder, Lunar Prospector, Genesis, Deep Impact, Propulsion (SEP), which enables substantial flexibility with Stardust, Kepler, GRAIL and MESSENGER [1]. -
Events: No General Meeting in April
The monthly newsletter of the Temecula Valley Astronomers Apr 2020 Events: No General Meeting in April. Until we can resume our monthly meetings, you can still interact with your astronomy associates on Facebook or by posting a message to our mailing list. General information: Subscription to the TVA is included in the annual $25 membership (regular members) donation ($9 student; $35 family). President: Mark Baker 951-691-0101 WHAT’S INSIDE THIS MONTH: <[email protected]> Vice President: Sam Pitts <[email protected]> Cosmic Comments Past President: John Garrett <[email protected]> by President Mark Baker Treasurer: Curtis Croulet <[email protected]> Looking Up Redux Secretary: Deborah Baker <[email protected]> Club Librarian: Vacant compiled by Clark Williams Facebook: Tim Deardorff <[email protected]> Darkness – Part III Star Party Coordinator and Outreach: Deborah Baker by Mark DiVecchio <[email protected]> Hubble at 30: Three Decades of Cosmic Discovery Address renewals or other correspondence to: Temecula Valley Astronomers by David Prosper PO Box 1292 Murrieta, CA 92564 Send newsletter submissions to Mark DiVecchio th <[email protected]> by the 20 of the month for Members’ Mailing List: the next month's issue. [email protected] Website: http://www.temeculavalleyastronomers.com/ Like us on Facebook Page 1 of 18 The monthly newsletter of the Temecula Valley Astronomers Apr 2020 Cosmic Comments by President Mark Baker One of the things commonly overlooked about Space related Missions is time, and of course, timing…!!! Many programs take a decade just to get them in place and off the ground, and many can take twice that long…just look at the James Webb Telescope!!! So there’s the “time” aspect of such endeavors…what about timing?? I mentioned last month that July is looking like a busy month for Martian Missions… here’s a refresher: 1) The NASA Mars 2020 rover Perseverance and its helicopter drone companion (aka Lone Ranger and Tonto, as I called them) is still on schedule.