Assessing the Mechanisms Underlying Visual Boundary Extension in Humans (Homo Sapiens), Rhesus Monkeys (Macaca Mulatta), and Capuchin Monkeys (Cebus Apella)
Total Page:16
File Type:pdf, Size:1020Kb
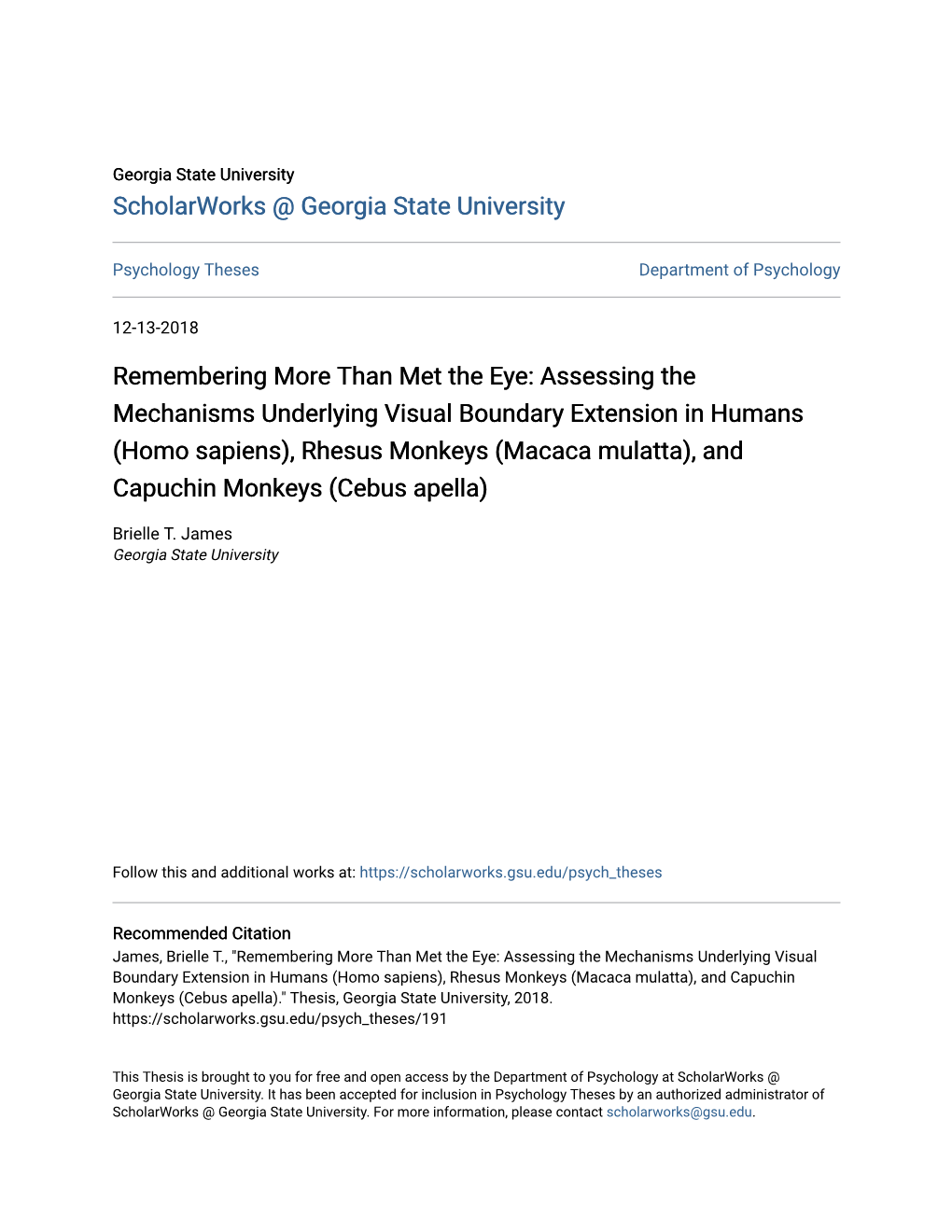
Load more
Recommended publications
-
Embodied Visual Recognition: Learning to Move for Amodal Perception
Embodied Visual Recognition Jianwei Yang1‹, Zhile Ren1‹, Mingze Xu2, Xinlei Chen3, David Crandall2, Devi Parikh1;3 Dhruv Batra1;3 1Georgia Institute of Technology, 2Indiana University, 3Facebook AI Research. https://www.cc.gatech.edu/˜jyang375/evr.html Abstract Passive visual systems typically fail to recognize objects in the amodal setting where they are heavily occluded. In contrast, humans and other embodied agents have the abil- ity to move in the environment, and actively control the viewing angle to better understand object shapes and se- mantics. In this work, we introduce the task of Embodied Visual Recognition (EVR): An agent is instantiated in a 3D Figure 1: The task of Embodied Visual Recognition: An environment close to an occluded target object, and is free agent is spawned close to an occluded target object in a 3D to move in the environment to perform object classification, environment, and asked for visual recognition, i.e., predict- amodal object localization, and amodal object segmenta- ing class label, amodal bounding box and amodal mask of tion. To address this, we develop a new model called Em- the target object. The agent is free to move around to aggre- bodied Mask R-CNN, for agents to learn to move strate- gate information for better visual recognition. gically to improve their visual recognition abilities. We conduct experiments using the House3D environment. Ex- perimental results show that: 1) agents with embodiment Recently, the dominant paradigm for object recognition (movement) achieve better visual recognition performance and amodal perception has been based on single image. than passive ones; 2) in order to improve visual recognition Though leveraging the advances of deep learning, visual abilities, agents can learn strategical moving paths that are systems still fail to recognize object and its shape from sin- different from shortest paths. -
Autolay: Benchmarking Amodal Layout Estimation for Autonomous Driving
2020 IEEE/RSJ International Conference on Intelligent Robots and Systems (IROS) October 25-29, 2020, Las Vegas, NV, USA (Virtual) AutoLay: Benchmarking amodal layout estimation for autonomous driving Kaustubh Mani∗1,2, N. Sai Shankar∗1, Krishna Murthy Jatavallabhula3,4, and K. Madhava Krishna1,2 1Robotics Research Center, KCIS, 2IIIT Hyderabad, 3Mila - Quebec AI Institute, Montreal, 4Université de Montréal Fig. 1: Amodal layout estimation is the task of estimating a semantic occupancy map in bird’s eye view, given a monocular image or video. The term amodal implies that we estimate occupancy and semantic labels even for parts of the world that are occluded in image space. In this work, we introduce AutoLay, a new dataset and benchmark for this task. AutoLay provides annotations in 3D, in bird’s eye view, and in image space. A sample annotated sequence (from the KITTI dataset [1]) is shown below. We provide high quality labels for sidewalks, vehicles, crosswalks, and lanes. We evaluate several approaches on sequences from the KITTI [1] and Argoverse [2] datasets. Abstract— Given an image or a video captured from a in [3]). Amodal perception, as studied by cognitive scientists, monocular camera, amodal layout estimation is the task of refers to the phenomenon of “imagining" or “hallucinating" predicting semantics and occupancy in bird’s eye view. The parts of the scene that do not result in sensory stimulation. In term amodal implies we also reason about entities in the scene that are occluded or truncated in image space. While the context of urban driving, we formulate the amodal layout several recent efforts have tackled this problem, there is a estimation task as predicting a bird’s eye view semantic map lack of standardization in task specification, datasets, and from monocular images that explicitly reasons about entities evaluation protocols. -
Four Theories of Amodal Perception
Four Theories of Amodal Perception Bence Nanay ([email protected]) Syracuse University, Department of Philosophy, 535 Hall of Languages Syracuse, NY 13244 USA Abstract perceive (Clarke, 1965; Strawson, 1979; Noë, 2004, p. 76). Do we perceive the entire cat? Or those parts of the cat that are visible, that is, a tailless cat? I do not intend to answer We are aware of those parts of a cat that are occluded behind any of these questions here. My question is not about what a fence. The question is how we represent these occluded we perceive but about the way in which we represent those parts of perceived objects: this is the problem of amodal parts of objects that are not visible to us. perception. I will consider four theories and compare their explanatory power: (i) we see them, (ii) we have non- Also, I need to emphasize that amodal perception is not a perceptual beliefs about them, (iii) we have immediate weird but rare subcase of our everyday awareness of the perceptual access to them and (iv) we visualize them. I point world. Almost all episodes of perception include an amodal out that the first three of these views face both empirical and component. For example, typically, only three sides of a conceptual objections. I argue for the fourth account, non-transparent cube are visible. The other three are not according to which we visualize the occluded parts of visible – we are aware of them ‘amodally’. The same goes perceived objects. Finally, I consider some important for houses or for any ordinary objects. -
Anamorphosis Implications AMODAL PERCEPTION
44 Amodal Perception Anamorphosis partly inspired by the “transactional approach” of philosopher John Dewey (who first saw the demon- The Ames demonstrations were not unprecedented, strations in 1946, and then corresponded with in the sense that they have much in common with Ames until 1951). Ames believed, as Dewey did, an historic artistic distortion technique called that we are not passive recipients of a given reality, anamorphosis, and to other perspective illusions but instead are active participants in a give-and-take employed in the design of theatrical sets. exchange (a “transaction”) in which split-second In anamorphic constructions, the image looks assumptions are made about the nature of reality. distorted when viewed frontally (as is customary) The demonstrations have also had lasting effects but correctly proportioned when seen from the on other aspects of culture. Even today, one or side (often indicated by a peephole). For example, more of the demonstrations are invariably men- Ames was well acquainted with the work of scien- tioned in textbooks on perception, and it is not tist and philosopher Hermann von Helmholtz, uncommon for one or more to appear in television who more than 50 years before had noted that an documentaries, video clips, cinematic special infinite variety of distorted rooms could be devised effects, or advertising commercials. that, from a monocular peephole, would nonethe- less seem to be normal. Roy R. Behrens Far in advance of Helmholtz, this same kind of visual distortion was used as early as 1485 by See also Magic and Perception; Object Perception; Leonardo da Vinci (and probably even earlier by Pictorial Depiction and Perception Chinese artists) as an offshoot of perspective. -
Visual Perception Glossary
Visual Perception Glossary Amodal perception The part of an object that is not visible because occlu- ded can be amodally perceived. Amodal perception is different and one step removed from modal percepti- on of real or illusory contours. Apparent motion When an object is presented at two different locati- ons after a brief time interval observers perceive mo- tion. The fi rst empirical investigation was carried out by Sigmund Exner . His aim, as well as the subsequent work by Max Wertheimer , was in establishing that motion was a basic sensation. Attention Refers to the selective processing of some aspect of information, while ignoring other information. The sudden onset of a stimulus can capture attention, but people can also exert some control on where they di- rect their attention. Bi-stable stimulus A particular stimulus that can produce two percepts over time, even though it is unchanged as a stimulus. An example is the Necker cube . Brightness Brightness refers to perception of how much light is coming from a given surface or object. A brighter objects refl ects more light than a less bright object. However perception of brightness is not fully deter- mined by luminance (see Illusory contours). 192 Visual Perception Glossary Cerebral lobe The cerebral cortex of the human brain is divided into four main lobes. Frontal (at the front), occipital (at the back), temporal (on the sides) and parietal (at the top). Consciousness Sorry this is too hard, your guess is as good as mine. Cortex The cortex is the outer layer of the brain . In most mammals the cortex is folded and this allows the surface to have a greater area given in the confi ned space available inside the skull. -
Amodal Detection of 3D Objects: Inferring 3D Bounding Boxes from 2D Ones in RGB-Depth Images
Amodal Detection of 3D Objects: Inferring 3D Bounding Boxes from 2D Ones in RGB-Depth Images Zhuo Deng Longin Jan Latecki Temple University, Philadelphia, USA [email protected], [email protected] Abstract localizations from monocular imagery [6, 13, 20, 3], and 3D object recognitions on CAD models [29, 27]. But these This paper addresses the problem of amodal perception works either rely on a huge number of ideal 3D graphics of 3D object detection. The task is to not only find object models by assuming the locations are known or are inclined localizations in the 3D world, but also estimate their phys- to fail in cluttered environments where occlusions are very ical sizes and poses, even if only parts of them are visible common while depth orders are uncertain. in the RGB-D image. Recent approaches have attempted The recent advent of Microsoft Kinect and similar sen- to harness point cloud from depth channel to exploit 3D sors alleviated some of these challenges, and thus enabled features directly in the 3D space and demonstrated the su- an exciting new direction of approaches to 3D object detec- periority over traditional 2.5D representation approaches. tion [18, 17, 12, 11, 19, 25, 24]. Equipped with an active We revisit the amodal 3D detection problem by sticking infrared structured light sensor, Kinect is able to provide to the 2.5D representation framework, and directly relate much more accurate depth locations of objects associated 2.5D visual appearance to 3D objects. We propose a novel with their visual appearances. The RGB-Depth detection 3D object detection system that simultaneously predicts ob- approaches can be roughly categorized into two groups ac- jects’ 3D locations, physical sizes, and orientations in in- cording to the way to formulate feature representations from door scenes. -
Abstracts of the Psychonomic Society — Volume 14 — November 2009 50Th Annual Meeting — November 19–22, 2009 — Boston, Massachusetts
Abstracts of the Psychonomic Society — Volume 14 — November 2009 50th Annual Meeting — November 19–22, 2009 — Boston, Massachusetts Papers 1–7 Friday Morning Scene Processing randomly positioned. When targets were systematically located within Grand Ballroom, Friday Morning, 8:00–9:35 scenes, search for them became progressively more efficient. Learning was abstracted away from bedrooms and transferred to a living room, Chaired by Carrick C. Williams, Mississippi State University where the target was on a sofa pillow. These contingencies were explicit and led to central tendency biases in memory for precise target positions. 8:00–8:15 (1) These results broaden the scope of conditions under which contextual Encoding and Visual Memory: Is Task Always Irrelevant? CAR- cuing operates and demonstrate for the first time that semantic memory RICK C. WILLIAMS, Mississippi State University—Although some plays a causal and independent role in the learning of associations be- aspects of encoding (e.g., presentation time) appear to have an effect tween objects in real-world scenes. on visual memories, viewing task (incidental or intentional encoding) does not. The present study investigated whether different encoding 9:20–9:35 (5) manipulations would impact visual memories equally for all objects Visual Memory: Confidence, Accuracy, and Recollection of Specific in a conjunction search (e.g., targets, color distractors, object category Details. GEOFFREY R. LOFTUS, University of Washington, MARK T. distractors, or distractors unrelated to the target). -
Between Perception and Imagination Philosophical and Cognitive Dilemmas
Between Perception and Imagination Philosophical and Cognitive Dilemmas By Edvard Avilés Meza Submitted to Central European University Department of Philosophy In partial fulfillment of the requirements for the degree of Master of Arts Supervisor: Tim Crane CEU eTD Collection Budapest, Hungary 2019 To my father, Jessie; my mother, Zoila and the memory of Dante Gazzolo CEU eTD Collection 2 Table of Contents Abstract .............................................................................................................................................................. 4 Introduction ...................................................................................................................................................... 5 Chapter 1. Imagining the External World ................................................................................................ 7 Constraining Imagination ............................................................................................................................. 8 Similarities and Differences between Perception and Mental Imagery ............................................... 11 Imagery-Perception Interaction ................................................................................................................. 14 Perceptual Incompleteness ......................................................................................................................... 16 Unconscious Imagination and ‘Mental Schimagery’ .............................................................................. -
Embodied Amodal Recognition: Learning to Move to Perceive Objects
Embodied Amodal Recognition: Learning to Move to Perceive Objects Jianwei Yang1‹ Zhile Ren1‹ Mingze Xu2 Xinlei Chen3 David J. Crandall2 Devi Parikh1,3 Dhruv Batra1,3 1Georgia Institute of Technology 2Indiana University 3Facebook AI Research Abstract Passive visual systems typically fail to recognize objects in the amodal setting where they are heavily occluded. In contrast, humans and other embodied agents have the abil- ity to move in the environment and actively control the view- ing angle to better understand object shapes and semantics. In this work, we introduce the task of Embodied Amodel Recognition (EAR): an agent is instantiated in a 3D envi- ronment close to an occluded target object, and is free to Figure 1: The task of Embodied Amodal Recognition: An move in the environment to perform object classification, agent is spawned close to an occluded target object in a amodal object localization, and amodal object segmenta- 3D environment, and performs amodal recognition, i.e., tion. To address this problem, we develop a new model predicting the category, amodal bounding box and amodal called Embodied Mask R-CNN for agents to learn to move mask of the target object. The agent is free to move around strategically to improve their visual recognition abilities. to aggregate information for better amodal recognition. We conduct experiments using a simulator for indoor en- vironments. Experimental results show that: 1) agents with embodiment (movement) achieve better visual recognition Recently, the dominant paradigm for object recognition performance than passive ones and 2) in order to improve and amodal perception has been based on single images. -
Movement, Affect, Sensation Brian Massumi
Movement, Affect, Sensation Brian Massumi l'HI LOSOPH y/,cu cw RA L sr.uDI ESj,sc I ENC E In Parablesfor 1/11: Virtual Brian Massumi views the body and media such as tclevision, film, and the Internet as proces.�� that operate on multiple registcrs of sensation beyond the reach of the reading techniques founded on the standard rhetorical and semiocic models. Rcnewing and assessing Wil liam James 's radical empiricism and Henri Berbrson's philosophy of perccption rhrough the filter of thc post war French philosophy ofDeleuze, Guattari, and Foucault, Massumi links a cultural logie of variati on to questions of movcment, affect, and sensation. lf such concepts are as fundamental as signs and significations, he ar6>ues, thcn a new ser of theoretical issues appear, and with them potential new paths for the wt:dding of scientific and cultural theory. "This is <lll extraordinary work of scholarship and thought, the most thorough going critique ami reformul.ition of the culture doctrine that l have read in years. Massumi 's prose has a dazzling and sometimes cutting clarity, and yet he bites into very big issues. People will be reading and talking about Pamblesfor thc Virtual for a loog time to come." -MEAGHAN MORRIS, L�11a11 U11i11ersity, I Ton)! Ko11g "Haw you been disappointed by books that promise to bring 'the body' or 'corporeality' back into nùture? Well, your luck i� about to changc. In this remarkabk book Bri:in Massumi transports us from the dicey intersection of movement and sens:ition,through insightful expJorations ofatTect and body image, to a creative reconfiguration of the 'nature-culture continuum.'The writing is cxperi mental and adventurous, a� one might expect from a writer who finds inventiveness to be the most distinctiw attribute of thinking. -
Amodal Completion, Perception and Visual Imagery
CLOTILDE CALABI Università degli Studi di Milano [email protected] Amodal Completion, Perception and Visual Imagery abstract Amodal completion typically occurs when we look at an object that is partially behind another object. Theorists often say that in such cases we are aware not only of the visible parts, but also, in some sense, of the occluded parts, because otherwise we could not have a perceptual experience of the object as continuing behind its occluder. Since no sense modality carries information about the occluded parts, this information is provided by other means. Amodal completion raises two questions. First, what is the mechanism involved? Second, what kind of experience do we have of the occluded parts? According to Nanay, the so-called Imagery Theory answers both questions. For this theory, information about the occluded parts is the product of a low level, vision specific, neural mechanism that takes place in the early vision processing areas of the brain. This mechanism provides a representation of the occluded parts and, as a result, the observer enjoys a quasi-sensory or quasi-perceptual conscious experience that is phenomenally similar to seeing those parts (as purportedly Perky has proved). In this paper I criticize Nanay’s answer to the second question. keywords Occlusion, amodal completion, visualization, mental imagery AmODAL COmplETION, PErcEPTION AND ViSUAL IMAGEry CLOTILDE CALABI Università degli Studi di Milano 1. Bence Nanay has recently brought back to our attention a famous experiment Amodal by Cheve West Perky, in which she tried to prove that perceiving and visualizing Completion and are phenomenally similar.1 The experiment consisted in the following. -
Embodied Amodal Recognition: Learning to Move to Perceive Objects
Embodied Amodal Recognition: Learning to Move to Perceive Objects Jianwei Yang1‹ Zhile Ren1‹ Mingze Xu2 Xinlei Chen3 David J. Crandall2 Devi Parikh1;3 Dhruv Batra1;3 1Georgia Institute of Technology 2Indiana University 3Facebook AI Research Abstract Passive visual systems typically fail to recognize objects in the amodal setting where they are heavily occluded. In contrast, humans and other embodied agents have the abil- ity to move in the environment and actively control the view- ing angle to better understand object shapes and semantics. In this work, we introduce the task of Embodied Amodel Recognition (EAR): an agent is instantiated in a 3D envi- ronment close to an occluded target object, and is free to Figure 1: The task of Embodied Amodal Recognition: An move in the environment to perform object classification, agent is spawned close to an occluded target object in a amodal object localization, and amodal object segmenta- 3D environment, and performs amodal recognition, i.e., tion. To address this problem, we develop a new model predicting the category, amodal bounding box and amodal called Embodied Mask R-CNN for agents to learn to move mask of the target object. The agent is free to move around strategically to improve their visual recognition abilities. to aggregate information for better amodal recognition. We conduct experiments using a simulator for indoor en- vironments. Experimental results show that: 1) agents with embodiment (movement) achieve better visual recognition Recently, the dominant paradigm for object recognition performance than passive ones and 2) in order to improve and amodal perception has been based on single images.