Experimental and Numerical Research on the Influence of Stern
Total Page:16
File Type:pdf, Size:1020Kb
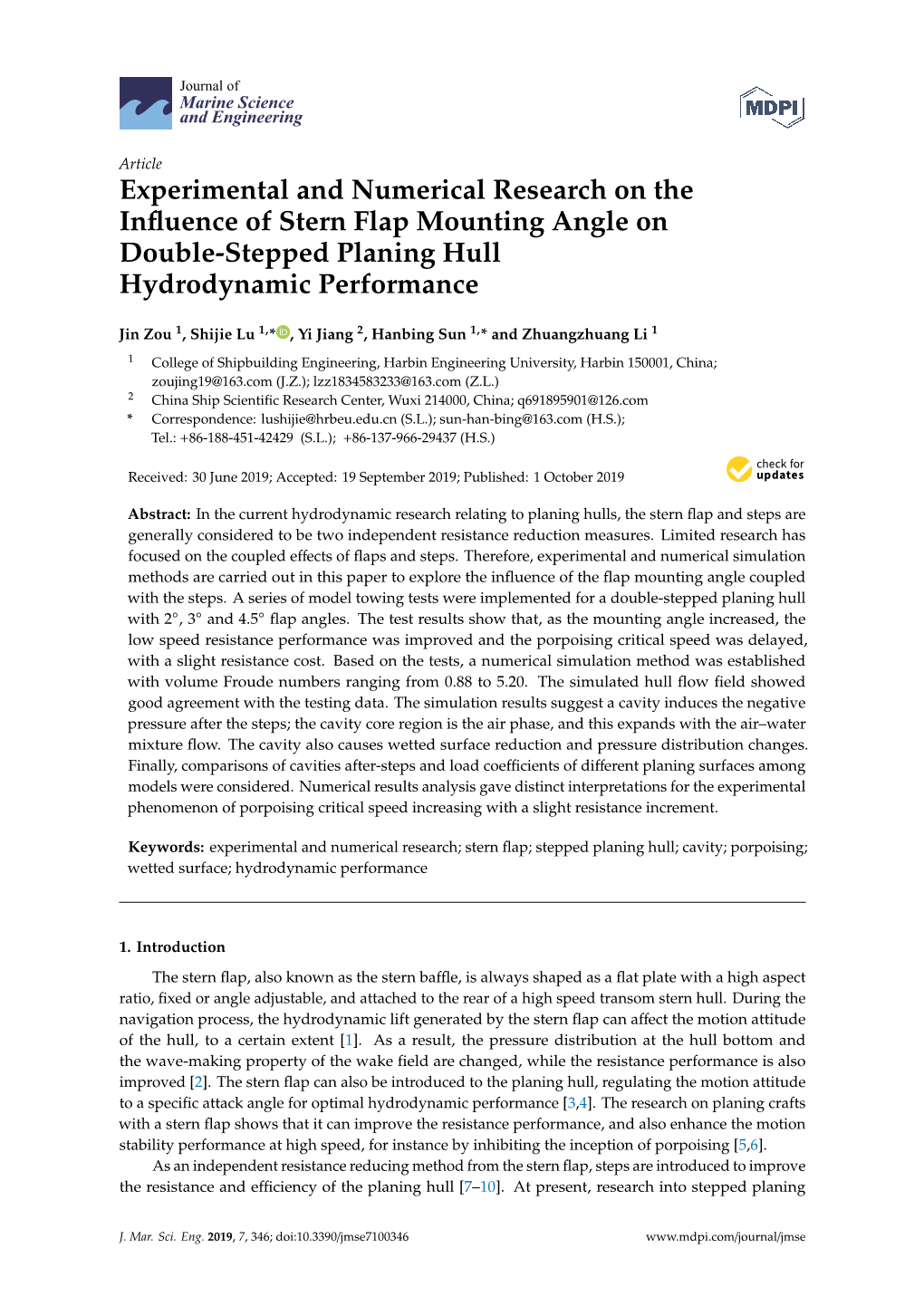
Load more
Recommended publications
-
Steering and Stabilisation Set a Course for Optimum Reliability and Performance
Marine Steering and stabilisation Set a course for optimum reliability and performance 1 Systems that keep vessels safely on course and comfortable in all conditions Since pioneering electro-hydraulic steering gear nearly a century ago, we continue to develop new systems for vessels ranging from large tankers to super yachts. Customers benefit from the world leading hydrodynamics expertise and the design resources of the Rolls-Royce rudder, steering gear, stabilisation and propulsion specialists, who cooperate to address and handle challenging projects and deliver system solutions. This minimises technical risk as well as maximising vessel performance. move Contents: Steering gear page 4 Promas page 10 Rudders page 12 Stabilisers page 18 Customer support page 22 movemake the right Steering gear Rotary vane steering gear for smaller vessels The SR series is designed with integrated frequency controlled pumps. General description Rolls-Royce supplies a complete range of steering gear, suitable for selection, alarm panels and rudder angle indicators or just a portion all types and sizes of ships. The products are designed as complete of this. The system is also prepared for interface to VDR, ships main steering systems with the actuator, power pack, steering control, alarm system, autopilot, joystick and DP when requested. Due to a alarm and rudder angle indicating system in mind, and can wide range of demands, great care has been taken from material therefore be delivered with complete control systems, including selection through construction, -
Coast Guard, DHS § 164.33
Coast Guard, DHS § 164.33 the United States unless no more than on a regular basis at least once every 12 hours before entering or getting un- three months. This drill must include derway, the following equipment has at a minimum the following: been tested: (1) Operation of the main steering (1) Primary and secondary steering gear from within the steering gear gear. The test procedure includes a vis- compartment. ual inspection of the steering gear and (2) Operation of the means of commu- its connecting linkage, and, where ap- nications between the navigating plicable, the operation of the following: bridge and the steering compartment. (i) Each remote steering gear control (3) Operation of the alternative power system. supply for the steering gear if the ves- (ii) Each steering position located on sel is so equipped. the navigating bridge. (92 Stat. 1471 (33 U.S.C. 1221 et seq.); 49 CFR (iii) The main steering gear from the 1.46(n)(4)) alternative power supply, if installed. (iv) Each rudder angle indicator in [CGD 77–183, 45 FR 18925, Mar. 24, 1980, as relation to the actual position of the amended by CGD 83–004, 49 FR 43466, Oct. 29, 1984] rudder. (v) Each remote steering gear control § 164.30 Charts, publications, and system power failure alarm. equipment: General. (vi) Each remote steering gear power No person may operate or cause the unit failure alarm. operation of a vessel unless the vessel (vii) The full movement of the rudder has the marine charts, publications, to the required capabilities of the and equipment as required by §§ 164.33 steering gear. -
Course Objectives Chapter 2 2. Hull Form and Geometry
COURSE OBJECTIVES CHAPTER 2 2. HULL FORM AND GEOMETRY 1. Be familiar with ship classifications 2. Explain the difference between aerostatic, hydrostatic, and hydrodynamic support 3. Be familiar with the following types of marine vehicles: displacement ships, catamarans, planing vessels, hydrofoil, hovercraft, SWATH, and submarines 4. Learn Archimedes’ Principle in qualitative and mathematical form 5. Calculate problems using Archimedes’ Principle 6. Read, interpret, and relate the Body Plan, Half-Breadth Plan, and Sheer Plan and identify the lines for each plan 7. Relate the information in a ship's lines plan to a Table of Offsets 8. Be familiar with the following hull form terminology: a. After Perpendicular (AP), Forward Perpendiculars (FP), and midships, b. Length Between Perpendiculars (LPP or LBP) and Length Overall (LOA) c. Keel (K), Depth (D), Draft (T), Mean Draft (Tm), Freeboard and Beam (B) d. Flare, Tumble home and Camber e. Centerline, Baseline and Offset 9. Define and compare the relationship between “centroid” and “center of mass” 10. State the significance and physical location of the center of buoyancy (B) and center of flotation (F); locate these points using LCB, VCB, TCB, TCF, and LCF st 11. Use Simpson’s 1 Rule to calculate the following (given a Table of Offsets): a. Waterplane Area (Awp or WPA) b. Sectional Area (Asect) c. Submerged Volume (∇S) d. Longitudinal Center of Flotation (LCF) 12. Read and use a ship's Curves of Form to find hydrostatic properties and be knowledgeable about each of the properties on the Curves of Form 13. Calculate trim given Taft and Tfwd and understand its physical meaning i 2.1 Introduction to Ships and Naval Engineering Ships are the single most expensive product a nation produces for defense, commerce, research, or nearly any other function. -
Boat Hull Hydrodynamics
Part I Boat Hull Hydrodynamics Introduction and Background Planing craft, particularly in the extensive worldwide boating industry, are the vastly dominant water craft type. “Planing” of a water craft occurs on accelerating to a sufficiently high speed. When such speed is initiated from rest in calm water, the hull bow angle is forced to increase upward, thereby increasing the angle of incidence of the boat bottom relative to the water surface. This incidence angle, interacting with the increasing boat speed, produces increased dynamic pressure on the boat bottom, which lifts the boat progressively further upward in elevation toward the level of the water surface. There is normally some overshoot: on reaching the level of the surface. The trim angle then decreases back toward equilibrium, with perhaps some oscillation, as the boat settles-in to steady planing on the calm water surface. Decisions required in the rational design of planing craft, particularly with regard to two aspects, are particularly difficult to conclude with precision: these aspects are planing speed and seaway motions (slamming). High speed and low seaway dynamics are the primary attributes sought in any new planing craft design undertaking, but they are conflicting. The characteristic most needed for low resistance, and consequently, high speed, is the weight located aft toward the stern of the boat. But weight aft tends to result in high impact acceleration when traversing waves. Further, weight aft can lead to “porpoising” instability, which is an oscillatory pitch motion in calm water accompanied by slamming oscillation. Conversely, weight forward results in reduced bow impact acceleration in waves, but also gives a long waterline, imposing higher resistance, and therefore reduced speed. -
RS Tera and Thank You for Choosing an RS Product
Rigging Manual V1 PLEASE FOLLOW RIGGING MANUAL IN THE CORRECT ORDER Contents 1. Introduction......................................................... 1 2. Technical data.................................................... 2 3. Commissioning................................................. 3 - 20 3.1 - Preparation.............................................................. 4 3.2 - Unpacking................................................................ 4 3.3 - Pack contents.......................................................... 4 - 5 3.4 - Adding the toestraps ................................................ 6 - 7 3.5 - Adding the toestrap elastic ...................................... 7 3.6 - Adding the rope handles .......................................... 8 3.7 - Adding the rear strop ............................................... 9 3.8 - Adding the bung ....................................................... 9 3.9 - Adding the righting lines .......................................... 10 3.10 - Adding ther painter ................................................ 10 3.11 - Rigging the mast .................................................... 11 - 12 3.12 - Stepping the mast .................................................. 12 3.13 - Rigging the boom ................................................... 13 - 17 3.14 - Rudder and daggerboard ...................................... 18 - 20 4. Sailing hints........................................................... 21 - 26 4.1 - Introduction.............................................................. -
Advanced Sailing – Lesson Plan Week 1
ADVANCED SAILING – LESSON PLAN WEEK 1 Date: June 1, 2019 # of Students: # of Boats: Resources: Desired Student Outcomes On Land: - Proficiency in sailing basics - Knowledge of boat parts and how to rig boat - Ability to answer basic seamanship questions On Water: - If not certified, re-certify skippers - Ability to perform both types of COBs recoveries - Ability to heave-to Chalk Talk Outline: - Review sailing basics – points of sail, sail trim, 4 basic maneuvers, parts of boat (see learn to sail Week 1 and Week 2 lesson plans) - Reassert importance of checking bilge, stopper knots, and safety equipment during rigging. - Reassert importance of securing mooring lines properly and opening scuppers during de-rigging - Answer questions on rigging, as needed. - Review basics on preparation, rules of the road, emergency responses, how to read wind, etc - Teach crew overboard (COB) procedures and recovery methods – Figure 8 and Quick Stop - Teach Heaving-To Activities and Drills: - Re-certifications for skippers - Practice both types of COB recovery methods - Practice heaving-to so there is no forward progress Parts of the Boat Contributor: Michael Egberts Main Halyard Mast Main Head Batten Jib Halyard Batten Jib Head Shroud Spreader Batten Mainsail Luff Main Batten Jib Lower Stay Jib Sheet Main Main Clew Main Foot Tack Jib Jib Clew Jib Foot Tack Mainsheet Bow Stern Hull Keel Gunwale 2016 RCYC Ideal 18 Handbook – Version 1.0 – May 25th, 2016 Page 11 of 28 Main Halyard Head Head Head Batten Luff Spinnaker Luff Mainsail Mainsail Tack Foot Spin Pole Jib Foot Tack Clew Tack Clew Main Hull Hull Stern Sheet Tiller Tiller Extension 2016 RCYC Ideal 18 Handbook – Version 1.0 – May 25th, 2016 Page 12 of 28 . -
Knowing Your Boat Means Knowing Its Wake
Knowing your boat means knowing its wake This is an illustrated guide to wave wake that expands upon the basics outlined in the low wake guide “Wake up? Slow Down”, which is available at: http://dpipwe.tas.gov.au/Documents/Guide-to-Low-Wave-Wake-Boating.pdf We all know ‘stow it – don’t throw it’ but what about what Bryce Courtenay’s Yowie said to the Dirty Rotten Rubbish Grumpkin: “not thinking is the same as not caring”? Here are 18 illustrations to help boaters think about their wake and its potential erosive impacts in sheltered, restricted or shallow waters. Environmental effects of wave wake In the open sea wave wake is likely to have little environmental effect since the waves are small compared to those that might occur naturally. It’s a very different matter in sheltered waters like riverine estuaries. The soft banks and beds of many Tasmanian waterways are vulnerable to damage from boat wake and prop wash. Typical easily-affected landforms include estuaries, inlets, lakes and lagoons; places where natural wave energy tends to be low. Banks of peat or mud, shallow lagoons or lakes with muddy beds and sandy deposits in upper estuaries are all areas of risk. Wake striking the banks can cause rapid and severe erosion, exposing the roots of vegetation and causing the banks to collapse. Wake impact and prop wash can also churn up fine bed sediments – the impact on bed and banks degrades the aquatic environment for plants and animals. In some popular boating and cruising areas, the effects of wake damage have been severe – for example, on the lower Gordon River, long stretches of bank have been washed away, with mature Huon pines and myrtles toppling into the river. -
International Optimist Class Rules CONTENTS
International Optimist Dinghy Association 2018 www.optiworld.org International Optimist Class Rules CONTENTS Page Rule 2 1 GENERAL 2 2. ADMINISTRATION 2 2.1 English language 2 2.2 Builders 3 2.3 World Sailing Class Fee 3 2.4 Registration and Measurement Certificate 4 2.5 Measurement 4 2.6 Measurement Instructions 5 2.7 Identification Marks 6 2.8 Advertising 6 3 CONSTRUCTIONAND MEASUREMENT RULES 6 3.1 General 6 3.2 Hull 6 3.2.1 Materials - GRP 7 3.2.2 Hull Measurement Rules 10 3.2.3 Hull Construction Details - GRP 12 3.2.4 Hull Construction Details - Wood and Wood/Epoxy (See Appendix A, p 27) 3.2.5 Not used 12 3.2.6 Fittings 13 3.2.7 Buoyancy 14 3.2.8 Weight 14 3.3 Daggerboard 16 3.4 Rudder and Tiller 19 3.5 Spars 19 3.5.2 Mast 20 3.5.3 Boom 21 3.5.4 Sprit 21 3.5.5 Running Rigging 22 4 ADDITIONAL RULES 5 (spare rule number) 23 6 SAIL 23 6.1 General 23 6.2 Sailmaker 6.3 Mainsail 6.4 Dimensions 25 6.5 Class Insignia, National Letters, Sail Numbers and Luff Measurement Band 26 6.6 Additional Rules (Sail) 27 APPENDIX A: Rules Specific to Wood and Wood/Epoxy Hulls. 29 PLANS: Index of current official plans. 30 Addendum - Information and References to World Sailing Advertising Code 1 1 GENERAL 1.1 The object of the class is to provide racing for young people at low cost. 1.2 The Optimist is a One-Design Class Dinghy. -
Boating Index
03/09/2016 CHAPTER P-2 - BOATING INDEX Page #200 Registration Information Required on Application for Vessel Number 2 #201 Dealer Licenses 3 #202 Expiration Date Decal 3 #203 Temporary Boat Permit 3 #204 Non-Resident Racing Boats 3 #205 Classification 3 #206 Measuring for Classification 4 #207 Lights 4 #208 Sound-Producing Devices 6 #209 Ventilation - Tank and Engine Spaces 6 #210 Backfire Flame Control 7 #211 Fire Extinguishers 7 #212 Personal Flotation Devises (PDF’s) 8 #213 Display of Capacity Information and Manufacturer Certification of 8 Compliance #214 Marine Sanitary Devices 10 #215 Buoys 10 #216 Scuba Diving 11 #217 River Use Restrictions 11 #218 Prohibited Operation 13 #219 Navigation and Rules of the Road 13 #220 Muffling and Sound Level 18 #221 Maneuvering and Warning Signals 19 #222 Collisions, Accidents, and Casualties 20 #223 Water Skis, Aquaplanes, Surfboards, Innertube, and Similar Devices 21 #224 Hull Identification Number 21 Basis and 23 Purpose Statement 1 CHAPTER P-2 - BOATING # 200 - REGISTRATION INFORMATION REQUIRED ON APPLICATION FOR VESSEL NUMBER 1. Persons applying to the Division for vessel number must provide the following registration information: a. Name and address of owner, including zip code b. Date of birth of owner c. State in which the vessel is or will be principally used d. Present registration number (if any) e. If vessel is registered in another state, give previous registration number and state f. Hull material: wood, metal, fiberglass, inflatable, or other g. Type of propulsion: inboard, outboard, inboard- outdrive, sail, or other h. Type of fuel: gasoline, diesel, electric, or other i. -
Chapter 7 Resistance and Powering of Ships
COURSE OBJECTIVES CHAPTER 7 7. RESISTANCE AND POWERING OF SHIPS 1. Define effective horsepower (EHP) conceptually and mathematically 2. State the relationship between velocity and total resistance, and velocity and effective horsepower 3. Write an equation for total hull resistance as a sum of viscous resistance, wave making resistance and correlation resistance. Explain each of these resistive terms. 4. Draw and explain the flow of water around a moving ship showing the laminar flow region, turbulent flow region, and separated flow region 5. Draw the transverse and longitudinal wave patterns when a displacement ship moves through the water 6. Define Reynolds number with a mathematical formula and explain each parameter in the Reynolds equation with units 7. Be qualitatively familiar with the following sources of ship resistance: a. Steering Resistance b. Air and Wind Resistance c. Added Resistance due to Waves d. Increased Resistance in Shallow Water 8. Read and interpret a ship resistance curve including humps and hollows 9. State the importance of naval architecture modeling for the resistance on the ship's hull 10. Define geometric and dynamic similarity 11. Write the relationships for geometric scale factor in terms of length ratios, speed ratios, wetted surface area ratios and volume ratios 12. Describe the law of comparison (Froude’s law of corresponding speeds) conceptually and mathematically, and state its importance in model testing 13. Qualitatively describe the effects of length and bulbous bows on ship resistance i 14. Be familiar with the momentum theory of propeller action and how it can be used to describe how a propeller creates thrust 15. -
Hull Classification Surveys
SC242 SC Arrangements for steering capability and 242 function on ships fitted with propulsion and (cont)(Jan 2011) steering systems other than traditional (Corr.1 arrangements for a ship’s directional control Aug 2011) (Chapter II-1, Regulations 29.1, 29.2.1, 29.3, 29.4, 29.6.1, 29.14, 28.3 and 30.2) (Rev.1 Apr Introduction 2016) The SOLAS requirements for steering gears have been established for ships having a traditional propulsion system and one rudder-type steering system. For ships fitted with alternative propulsion and steering systems without rudder, such as but not limited to azimuthing propulsors or water jet propulsion systems, SOLAS Regulations II-1/29.1, 29.2.1, 29.3, 29.4, 29.6.1, 29.14, 28.3 and 30.2 are to be interpreted as follows, except 29.14, which is limited to the steering systems having a certain steering capability due to vessel speed also in case propulsion power has failed. Definition: “Steering system” is ship’s directional control system, including main steering gear, auxiliary steering gear, steering gear control system and rudder if any. Regulation 29.1 29.1 Unless expressly provided otherwise, every ship shall be provided with a main steering gear and an auxiliary steering gear to the satisfaction of the administration. The main steering gear and the auxiliary steering gear shall be so arranged that the failure of one of them will not render the other one inoperative. Note: 1) This UI is to be uniformly implemented by IACS Members and Associates for propulsion and steering systems other than traditional arrangements for a ship’s directional control: a) when an application for certification of non traditional steering systems is dated on or after 1 January 2012; or b) which are installed in a new ship for which the date of contract for construction is on or after 1 January 2012. -
48 Kanter 48
PAINE 48 ALUMINUM OFFSHORE CRUISER DIMENSIONS LOA: 47' 11" LWL: 41' 4" BEAM 14' 2" DRAFT 7' 2" DISPLACEMENT 35,000 lbs BALLAST 11,825 lbs SAIL AREA 1097 sq ft D/L RATIO 222 SA/D RATIO 16.41 A TRADITIONAL PROFILE WITH A TALL AND POWERFUL RIG. Kanter Yachts of St. Thomas, Ontario is well along on the construction of this welded aluminum cruiser for a Colorado owner. The PAINE 48 is intended for fast offshore cruising in the safety of an all metal hull. A powerful rig is balanced by a moderate draft bulbed keel for controllable speed in all conditions. The rudder is skeg supported with the propeller in an aperture where it is less likely to snag lobster pot lines or other nautical flotsam. A Hydrovane self steerer will be fitted and for this reason the hull and appendages are perfectly balanced to reduce the loads on the small Hydrovane rudder. VOLVO D275 LWL LWL DWL DWL CRASH TANK DATUM FOR VERTICAL DIMENSIONS IS DWL top of finished sole 11-1/2" top of finished sole TOP OF FINISHED SOLE -11 1/2" ~3/8"~ ~3/8"~ 174 US. GALS. 182 US. GALS. DIESEL FUEL FRESH WATER 11500 POUNDS POURED-IN LEAD BALLAST WASHER/DRYER ELECTRICAL PANEL FREEZER REF'R The owner has been very specific about his requirements for the interior. Unlike most center cockpit designs, which step down the areas adjacent the cockpit, the cabin sole in this design is on a single level. Thus there is no possibility of tripping as one moves forward or aft in the yacht.