Is the Locomotion of Crawling Human Infants Different from Other Quadruped Mammals?
Total Page:16
File Type:pdf, Size:1020Kb
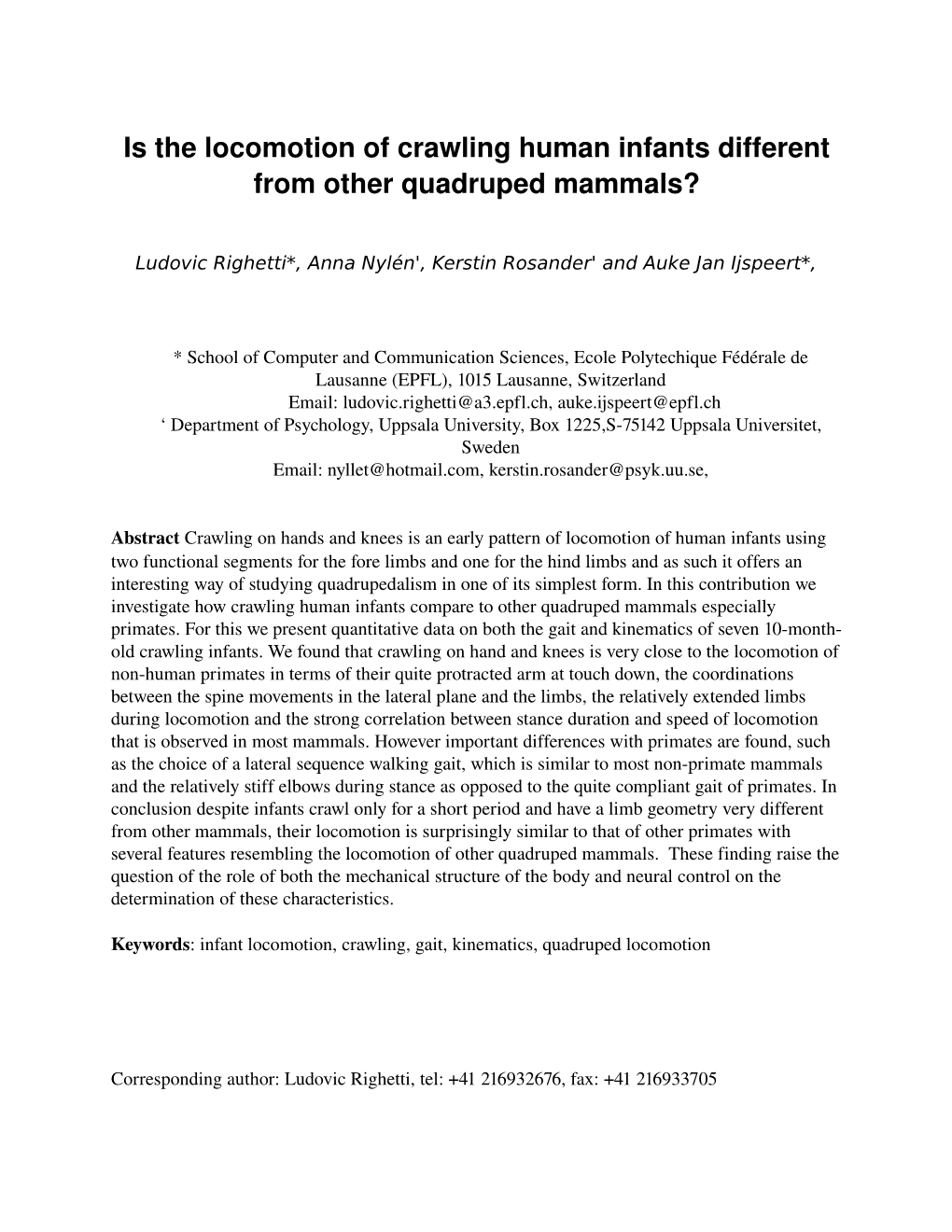
Load more
Recommended publications
-
Amphibious Fishes: Terrestrial Locomotion, Performance, Orientation, and Behaviors from an Applied Perspective by Noah R
AMPHIBIOUS FISHES: TERRESTRIAL LOCOMOTION, PERFORMANCE, ORIENTATION, AND BEHAVIORS FROM AN APPLIED PERSPECTIVE BY NOAH R. BRESSMAN A Dissertation Submitted to the Graduate Faculty of WAKE FOREST UNIVESITY GRADUATE SCHOOL OF ARTS AND SCIENCES in Partial Fulfillment of the Requirements for the Degree of DOCTOR OF PHILOSOPHY Biology May 2020 Winston-Salem, North Carolina Approved By: Miriam A. Ashley-Ross, Ph.D., Advisor Alice C. Gibb, Ph.D., Chair T. Michael Anderson, Ph.D. Bill Conner, Ph.D. Glen Mars, Ph.D. ACKNOWLEDGEMENTS I would like to thank my adviser Dr. Miriam Ashley-Ross for mentoring me and providing all of her support throughout my doctoral program. I would also like to thank the rest of my committee – Drs. T. Michael Anderson, Glen Marrs, Alice Gibb, and Bill Conner – for teaching me new skills and supporting me along the way. My dissertation research would not have been possible without the help of my collaborators, Drs. Jeff Hill, Joe Love, and Ben Perlman. Additionally, I am very appreciative of the many undergraduate and high school students who helped me collect and analyze data – Mark Simms, Tyler King, Caroline Horne, John Crumpler, John S. Gallen, Emily Lovern, Samir Lalani, Rob Sheppard, Cal Morrison, Imoh Udoh, Harrison McCamy, Laura Miron, and Amaya Pitts. I would like to thank my fellow graduate student labmates – Francesca Giammona, Dan O’Donnell, MC Regan, and Christine Vega – for their support and helping me flesh out ideas. I am appreciative of Dr. Ryan Earley, Dr. Bruce Turner, Allison Durland Donahou, Mary Groves, Tim Groves, Maryland Department of Natural Resources, UF Tropical Aquaculture Lab for providing fish, animal care, and lab space throughout my doctoral research. -
Gait Analysis in Uner Tan Syndrome Cases with Key Symptoms of Quadrupedal Locomotion, Mental Impairment, and Dysarthric Or No Speech
Article ID: WMC005017 ISSN 2046-1690 Gait Analysis in Uner Tan Syndrome Cases with Key Symptoms of Quadrupedal Locomotion, Mental Impairment, and Dysarthric or No Speech Peer review status: No Corresponding Author: Submitting Author: Prof. Uner Tan, Senior Researcher, Cukurova University Medical School , Cukurova University, Medical School, Adana, 01330 - Turkey Article ID: WMC005017 Article Type: Research articles Submitted on: 09-Nov-2015, 01:39:27 PM GMT Published on: 10-Nov-2015, 08:43:08 AM GMT Article URL: http://www.webmedcentral.com/article_view/5017 Subject Categories: NEUROSCIENCES Keywords: Uner Tan syndrome, quadrupedal locomotion, ataxia, gait, lateral sequence, diagonal sequence, evolution, primates How to cite the article: Tan U. Gait Analysis in Uner Tan Syndrome Cases with Key Symptoms of Quadrupedal Locomotion, Mental Impairment, and Dysarthric or No Speech. WebmedCentral NEUROSCIENCES 2015;6(11):WMC005017 Copyright: This is an open-access article distributed under the terms of the Creative Commons Attribution License (CC-BY), which permits unrestricted use, distribution, and reproduction in any medium, provided the original author and source are credited. Source(s) of Funding: None Competing Interests: None Additional Files: Illustration 1 ILLUSTRATION 2 ILLUSTRATION 3 ILLUSTRATION 4 ILLUSTRATION 5 ILLUSTRATION 6 WebmedCentral > Research articles Page 1 of 14 WMC005017 Downloaded from http://www.webmedcentral.com on 10-Nov-2015, 08:43:10 AM Gait Analysis in Uner Tan Syndrome Cases with Key Symptoms of Quadrupedal Locomotion, Mental Impairment, and Dysarthric or No Speech Author(s): Tan U Abstract sequence, on the lateral and on the diagonals.” This child typically exhibited straight legs during quadrupedal standing. Introduction: Uner Tan syndrome (UTS) consists of A man with healthy legs walking on all fours was quadrupedal locomotion (QL), impaired intelligence discovered by Childs [3] in Turkey, 1917. -
Human Hands-And-Knees Crawling Movement Analysis Based on Time-Varying Synergy and Synchronous Synergy Theories
Mathematical Biosciences and Engineering, 16(4): 2492–2513. DOI: 10.3934/mbe.2019125 Received: 24 December 2018 Accepted: 06 March 2019 Published: 22 March 2019 http://www.aimspress.com/journal/MBE Research article Human hands-and-knees crawling movement analysis based on time-varying synergy and synchronous synergy theories Teng Li , Xiang Chen *, Shuai Cao , Xu Zhang and Xun Chen Department of Electronic Science and Technology, University of Science and Technology of China, Hefei 230026, Anhui, P.R. China * Correspondence: Email: [email protected]. Abstract: This paper aims to investigate human hands-and-knees crawling movement from the aspect of synchronous (SYN) and time-varying (TV) muscle synergy analysis. Nine healthy children and 11 children with cerebral palsy were recruited. During hands-and-knees crawling, surface electromyography (sEMG) signals from 12 main muscles of upper limbs and trunk were recorded, and muscle synergies were extracted based on TV synergy and SYN synergy theories. From the perspectives of repeatability, symmetry and similarity, the abilities of these two types of synergies to characterize crawling movement and to distinguish normal and abnormal crawling were explored. We found that: First, SYN synergy is better than TV synergy in depicting the body symmetry during crawling movement. However, TV synergy is more suitable than SYN synergy for distinguishing normal and abnormal crawling from the perspective of symmetry. Second, the abilities of SYN synergy and TV synergy in depicting the crawling repeatability are not comparable, and both have the potential to depict the crawling abnormality from the perspective of repeatability. Third, from the angle of inter-subject similarity, SYN synergy has the potential to describe the abnormal crawling pattern. -
Kinematic and Gait Similarities Between Crawling Human Infants and Other Quadruped Mammals
ORIGINAL RESEARCH ARTICLE published: 09 February 2015 doi: 10.3389/fneur.2015.00017 Kinematic and gait similarities between crawling human infants and other quadruped mammals Ludovic Righetti 1*, Anna Nylén2, Kerstin Rosander 2 and Auke Jan Ijspeert 3 1 Autonomous Motion Department, Max-Planck Institute for Intelligent Systems, Tübingen, Germany 2 Uppsala Child and Baby Lab, Department of Psychology, Uppsala University, Uppsala, Sweden 3 Biorobotics Laboratory, Interfaculty Institute of Bioengineering, School of Engineering, École Polytechnique Fédérale de Lausanne, Lausanne, Switzerland Edited by: Crawling on hands and knees is an early pattern of human infant locomotion, which offers Uner Tan, Cukurova University, Turkey an interesting way of studying quadrupedalism in one of its simplest form. We investigate Reviewed by: how crawling human infants compare to other quadruped mammals, especially primates. Héctor Alberto González-Usigli, Instituto Mexicano del Seguro Social, We present quantitative data on both the gait and kinematics of seven 10-month-old crawl- Mexico ing infants. Body movements were measured with an optoelectronic system giving precise Katya Kotschet, St Vincent’s Hospital, data on 3-dimensional limb movements. Crawling on hands and knees is very similar to the Australia locomotion of non-human primates in terms of the quite protracted arm at touch-down, the *Correspondence: coordination between the spine movements in the lateral plane and the limbs, the relatively Ludovic Righetti, Autonomous Motion extended limbs during locomotion and the strong correlation between stance duration and Department, Max-Planck Institute for Intelligent Systems, Paul-Erlich Str. speed of locomotion. However, there are important differences compared to primates, 15, Tübingen 72076, Germany such as the choice of a lateral-sequence walking gait, which is similar to most non-primate e-mail: ludovic.righetti@tuebingen. -
Locomotion of Reptiles" Will Be of Interest to a Wide Range of Readers
REVIEW A RTICLES Editorial note. We are trying to introduce a "new look" to the BHS Bulletin, and one of our plans is to commission articles by well-known zoologists summarising recent advances in their area of expertise, as they relate to herpetology. We are particularly pleased that Professor McNeill Alexander of Leeds University agreed to write the first of these. We hope that this masterly summary of "Locomotion of Reptiles" will be of interest to a wide range of readers. Roger Meek and Roger Avery, Co-Editors. Locomotion of Reptiles R. McNEILL ALEXANDER Institute for Integrative and Comparative Biology, University of Leeds, Leeds LS2 9JT, UK. [email protected] ABSTRACT – Reptiles run, crawl, climb, jump, glide and swim. Exceptional species run on the surface of water or “swim” through dry sand. This paper is a short summary of current knowledge of all these modes of reptilian locomotion. Most of the examples refer to lizards or snakes, but chelonians and crocodilians are discussed briefly. Extinct reptiles are omitted. References are given to scientific papers that provide more detailed information. INTRODUCTION the body and the leg joints much straighter, as in his review is an attempt to explain briefly elephants. The bigger an animal is, the harder it Tthe many different modes of locomotion that is for them to support the weight of the body in a reptiles use. Some of the information it gives lizard-like stance. Imagine two reptiles of exactly has been known for many years, but many of the the same shape, one twice as long as the other. -
Alexander 2013 Principles-Of-Animal-Locomotion.Pdf
.................................................... Principles of Animal Locomotion Principles of Animal Locomotion ..................................................... R. McNeill Alexander PRINCETON UNIVERSITY PRESS PRINCETON AND OXFORD Copyright © 2003 by Princeton University Press Published by Princeton University Press, 41 William Street, Princeton, New Jersey 08540 In the United Kingdom: Princeton University Press, 3 Market Place, Woodstock, Oxfordshire OX20 1SY All Rights Reserved Second printing, and first paperback printing, 2006 Paperback ISBN-13: 978-0-691-12634-0 Paperback ISBN-10: 0-691-12634-8 The Library of Congress has cataloged the cloth edition of this book as follows Alexander, R. McNeill. Principles of animal locomotion / R. McNeill Alexander. p. cm. Includes bibliographical references (p. ). ISBN 0-691-08678-8 (alk. paper) 1. Animal locomotion. I. Title. QP301.A2963 2002 591.47′9—dc21 2002016904 British Library Cataloging-in-Publication Data is available This book has been composed in Galliard and Bulmer Printed on acid-free paper. ∞ pup.princeton.edu Printed in the United States of America 1098765432 Contents ............................................................... PREFACE ix Chapter 1. The Best Way to Travel 1 1.1. Fitness 1 1.2. Speed 2 1.3. Acceleration and Maneuverability 2 1.4. Endurance 4 1.5. Economy of Energy 7 1.6. Stability 8 1.7. Compromises 9 1.8. Constraints 9 1.9. Optimization Theory 10 1.10. Gaits 12 Chapter 2. Muscle, the Motor 15 2.1. How Muscles Exert Force 15 2.2. Shortening and Lengthening Muscle 22 2.3. Power Output of Muscles 26 2.4. Pennation Patterns and Moment Arms 28 2.5. Power Consumption 31 2.6. Some Other Types of Muscle 34 Chapter 3. -
Human Crawling Performance and Technique Revealed by Inertial Measurement Units ⇑ Rachel V
Journal of Biomechanics 84 (2019) 121–128 Contents lists available at ScienceDirect Journal of Biomechanics journal homepage: www.elsevier.com/locate/jbiomech www.JBiomech.com Human crawling performance and technique revealed by inertial measurement units ⇑ Rachel V. Vitali , Stephen M. Cain, Steven P. Davidson, Noel C. Perkins Department of Mechanical Engineering, University of Michigan, Ann Arbor, MI, USA article info abstract Article history: Human crawling performance and technique are of broad interest to roboticists, biomechanists, and mil- Accepted 15 December 2018 itary personnel. This study explores the variables that define crawling performance in the context of an outdoor obstacle course used by military organizations worldwide to evaluate the effects of load and per- sonal equipment on warfighter performance. Crawling kinematics, measured from four body-worn iner- Keywords: tial measurement units (IMUs) attached to the upper arms and thighs, are recorded for thirty-three Inertial measurement units participants. The IMU data is distilled to four metrics of crawling performance; namely, crawl speed, Crawling crawl stride time, ipsilateral limb coordination, and contralateral limb coordination. We hypothesize that Limb coordination higher performance (as identified by higher crawl speeds) is associated with more coordinated limbs and lower stride times. A cluster analysis groups participants into high and low performers exhibiting statis- tically significant differences across the four performance metrics. In particular, high performers exhibit superior limb coordination associated with a ‘‘diagonal gait” in which contralateral limbs move largely in-phase to produce faster crawl speeds and shorter crawl stride times. In contrast, low performers crawl at slower speeds with longer crawl stride times and less limb coordination. -
Gross Motor Skills Practical Advice and Activity Ideas to Encourage the Development of Gross Motor Skills
Children and Young People’s Occupational Therapy Service Gross Motor Skills Practical advice and activity ideas to encourage the development of Gross Motor skills. Follow us on Twitter @NHSaaa Find us on Facebook at www.facebook.com/nhsaaa Visit our website: www.nhsaaa.net All our publications are available in other formats Contents page Introduction 3 Planning and Organisation 5 Bilateral Integration 8 Core Stability 12 Spatial awareness 14 Ball skills 17 General gross motor activities 20 Useful resources and websites 23 2 Introduction This booklet has been designed to provide practical ideas and activities to help support your child/ young person to develop their gross motor skills. All references to child / children mean either a child or young person. Gross motor skills are large movements your child makes with their arms, legs, feet, or entire body. For example; crawling, running and jumping are gross motor skills. Gross motor skills can be challenging for many children for a number of reasons: • Lack of experience or practice. • Difficulty using two sides of the body together (bilateral integration). • Reduced balance caused by poor core stability. • Difficulty planning and organising themselves to follow instructions. • Poor spatial (space) awareness skills. In many cases, supporting a child on a regular basis to practice gross motor activities should encourage their skills to improve. 3 Games and activities can be introduced to your child at any time throughout the day. Try to create lots of opportunities as this can be very helpful for your child’s gross motor development. The booklet also contains other useful resources; local authority websites, active school co-ordinators, clubs. -
A Hybrid Vehicle for Aerial and Terrestrial Locomotion
A HYBRID VEHICLE FOR AERIAL AND TERRESTRIAL LOCOMOTION by Richard J. Bachmann Submitted in partial fulfillment of the requirements for the degree of Doctor of Philosophy Dissertation Advisor: Dr. Roger D. Quinn Department of Mechanical Engineering Case Western Reserve University May, 2008 CASE WESTERN RESERVE UNIVERSITY SCHOOL OF GRADUATE STUDIES We hereby approve the thesis/dissertation of _____________________________________________________ candidate for the ______________________degree *. (signed)_______________________________________________ (chair of the committee) ________________________________________________ ________________________________________________ ________________________________________________ ________________________________________________ ________________________________________________ (date) _______________________ *We also certify that written approval has been obtained for any proprietary material contained therein. Table of Contents Section Page Chapter 1 Introduction..................................................................................................1 1.1 Motivation.............................................................................................................2 1.1.1 Chemical or Biological Weapon Source Localization....................................2 1.1.2 Ad hoc Sensor Network Deployment .............................................................2 1.1.3 Search and Rescue..........................................................................................3 1.1.4 Hazardous -
Experimental Study of Military Crawl As a Special Type of Human Quadripedal Automatic Locomotion
applied sciences Article Experimental Study of Military Crawl as a Special Type of Human Quadripedal Automatic Locomotion Dmitry Skvortsov 1,2,* , Victor Anisimov 2,3,* and Alina Aizenshtein 2,4 1 Federal Scientific and Clinical Center for Specialized Medical Assistance and Medical Technologies of the Federal Medical Biological Agency, 115682 Moscow, Russia 2 Dmitry Rogachev National Medical Research Center of Pediatric Hematology, Oncology and Immunology, 117997 Moscow, Russia; [email protected] 3 Faculty of Biology, Lomonosov Moscow State University, 119991 Moscow, Russia 4 Federal Center of Brain and Neurotechnology, 117997 Moscow, Russia * Correspondence: [email protected] (D.S.); [email protected] (V.A.); Tel.: +7-916-692-5419 (D.S.); +7-926-519-6801 (V.A.) Featured Application: The results of this study can be useful for further research into features related to the development of military crawl locomotion in groups of different ages, and for un- derstanding the development of this type of locomotion in ontogenesis. In rehabilitation clinics, it is possible to compare patients’ indicators with the norm registered in this study (which could be further expanded), to assess the degree of violations in the motor control system. Abstract: The biomechanics of military crawl locomotion is poorly covered in scientific literature so far. Crawl locomotion may be used as a testing procedure which allows for the detection of not only obvious, but also hidden locomotor dysfunctions. The aim of the study was to investigate the biomechanics of crawling among healthy adult participants. Eight healthy adults aged 15–31 Citation: Skvortsov, D.; Anisimov, V.; (four women and four men) were examined by means of a 3D kinematic analysis with Optitrack Aizenshtein, A. -
Master Thesis Project Human-Like Crawling for Humanoid Robots
Master Thesis Project Human-like Crawling for Humanoid Robots - Gait Evaluation on the NAO robot Author: Andreas Aspernäs Supervisor: Johan Hagelbäck Examiner: Welf Löwe Semester: VT 2018 Course Code: 4DV50E Subject: Computer Science Abstract Human-robot interaction (HRI) is the study of how we as humans interact and communicate with robots and one of its subfields is working on how we can improve the collaboration between humans and robots. We need robots that are more user friendly and easier to understand and a key aspect of this is human-like movements and behavior. This project targets a specific set of motions called locomotion and tests them on the humanoid NAO robot. A human-like crawling gait was developed for the NAO robot and compared to the built-in walking gait through three kinds of experiments. The first one to compare the speed of the two gaits, the second one to estimate their sta- bility, and the third to examine how long they can operate by measuring the power consumption and temperatures in the joints. The results showed the robot was significantly slower when crawling compared to walking, and when still the robot was more stable while standing than on all-fours. The power consumption remained essentially the same, but the crawling gait ended up having a shorter operational time due to higher temperature increase in the joints. While the crawling gait has benefits of having a lower profile then the walking gait and could therefore more easily pass under low hanging obsta- cles, it does have major issues that needs to be addressed to become a viable solution. -
Uner Tan Syndrome: History, Clinical Evaluations, Genetics, and the Dynamics of Human Quadrupedalism Uner Tan*,1
78 The Open Neurology Journal, 2010, 4, 78-89 Open Access Uner Tan Syndrome: History, Clinical Evaluations, Genetics, and the Dynamics of Human Quadrupedalism Uner Tan*,1 Department of Physiology, Çukurova University, Medical School, 01330 Adana, Turkey Abstract: This review includes for the first time a dynamical systems analysis of human quadrupedalism in Uner Tan syndrome, which is characterized by habitual quadrupedalism, impaired intelligence, and rudimentary speech. The first family was discovered in a small village near Iskenderun, and families were later found in Adana and two other small vil- lages near Gaziantep and Canakkale. In all the affected individuals dynamic balance was impaired during upright walking, and they habitually preferred walking on all four extremities. MRI scans showed inferior cerebellovermian hypoplasia with slightly simplified cerebral gyri in three of the families, but appeared normal in the fourth. PET scans showed a de- creased glucose metabolic activity in the cerebellum, vermis and, to a lesser extent the cerebral cortex, except for one pa- tient, whose MRI scan also appeared to be normal. All four families had consanguineous marriages in their pedigrees, suggesting autosomal recessive transmission. The syndrome was genetically heterogeneous. Since the initial discoveries more cases have been found, and these exhibit facultative quadrupedal locomotion, and in one case, late childhood onset. It has been suggested that the human quadrupedalism may, at least, be a phenotypic example of reverse evolution. From the viewpoint of dynamic systems theory, it was concluded there may not be a single factor that predetermines human quadrupedalism in Uner Tan syndrome, but that it may involve self-organization, brain plasticity, and rewiring, from the many decentralized and local interactions among neuronal, genetic, and environmental subsystems.