How Coverage Influences Thermodynamic and Kinetic Isotope Effects for H2/D2 Dissociative Adsorption on Transition Metals
Total Page:16
File Type:pdf, Size:1020Kb
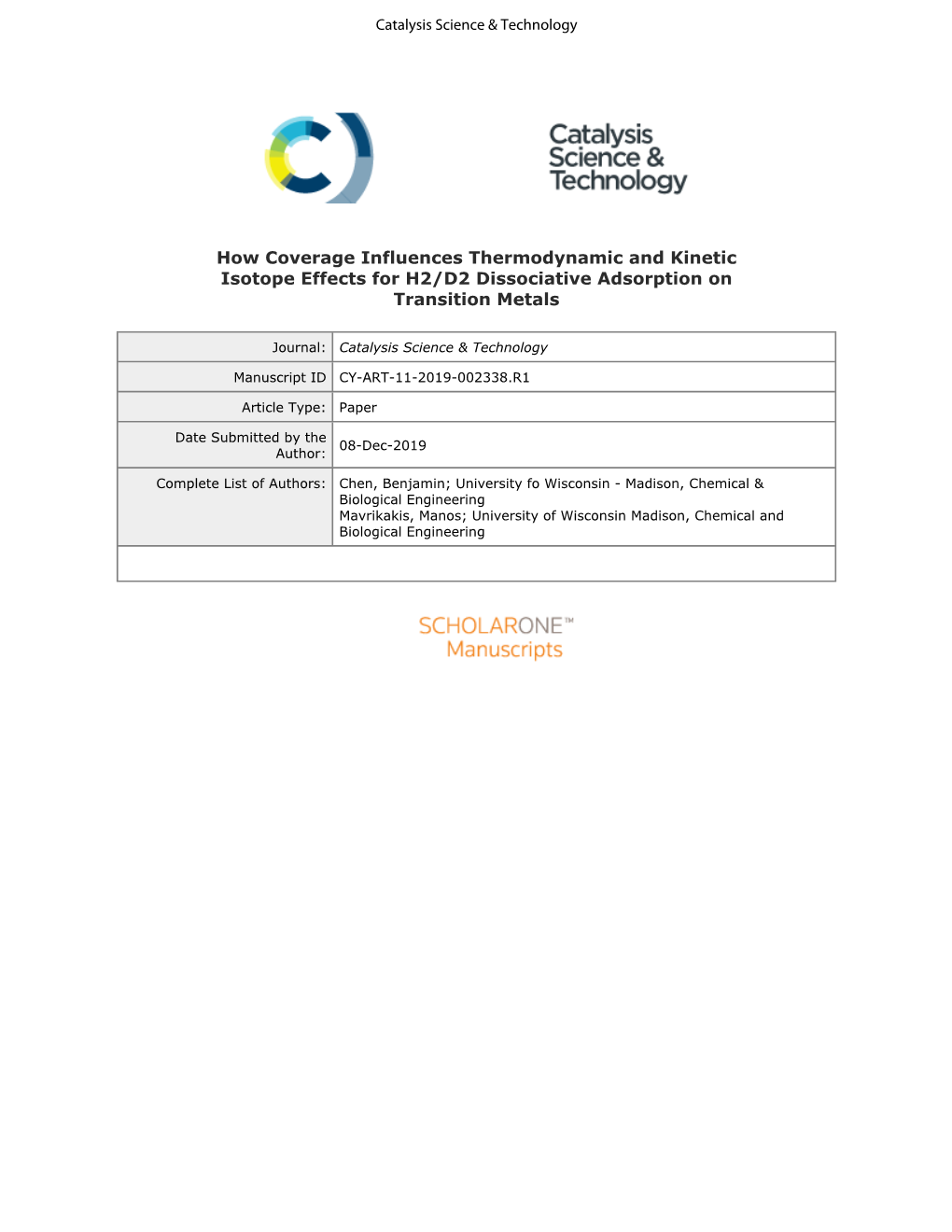
Load more
Recommended publications
-
The Kinetics of Elementary Thermal Reactions in Heterogene- Ous Catalysis
1 The kinetics of elementary thermal reactions in heterogene- 2 ous catalysis 3 G. Barratt Park1,2, Theofanis Kitsopoulos2,5,6, Dmitriy Borodin1, Kai Golibrzuch2, Jannis 4 Neugebohren1, Daniel J. Auerbach2, Charles T. Campbell4 & Alec M. Wodtke1,2,3* 5 1Institute for Physical Chemistry, Georg-August University of Goettingen, Tammannstraße 6, 6 37077 Göttingen, Germany. 7 2Department of Dynamics at Surfaces, Max Planck Institute for Biophysical Chemistry, Am 8 Faßberg 11, 37077 Göttingen, Germany. 9 3International Center for Advanced Studies of Energy Conversion, Georg-August University 10 of Goettingen, Tammannstraße 6, 37077 Göttingen, Germany. 11 4 Department of Chemistry, University of Washington, Seattle, WA 98195-1700, USA 12 5 Department of Chemistry, University of Crete, Heraklion, Greece 13 6 Institute of Electronic Structure and Laser – FORTH, Heraklion, Greece 14 15 *email: [email protected] 16 17 KEYWORDS: Ion-imaging, velocity-resolved kinetics, CO oxidation, heterogeneous catalysis, 18 slice ion-imaging, molecular beams, surface chemistry. 19 20 1 21 Abstract 22 The kinetics of elementary reactions is fundamental to our understanding of catalysis. Just as 23 micro-kinetic models of atmospheric chemistry provided the predictive power that led to the 24 Montreal protocol reversing loss of stratospheric ozone, pursuing a micro-kinetic approach to 25 heterogeneous catalysis has tremendous potential for societal impact. However, developing this 26 approach for catalysis faces great challenges. Methods for measuring rate constants are quite 27 limited and present predictive theoretical methods remain largely un-validated. This paper pre- 28 sents a short perspective on recent experimental advances in our ability to measure the rates of 29 elementary reactions at surfaces that rely on a stroboscopic pump-probe concept for neutral 30 matter. -
I IMPROVING PALLADIUM CATALYSIS with SELF
IMPROVING PALLADIUM CATALYSIS WITH SELF-ASSEMBLED MONOLAYERS by STEPHEN T. MARSHALL B.S., Carnegie Mellon University, 2005 A thesis submitted to the Faculty of the Graduate School of the University of Colorado in partial fulfillment of the requirement for the degree of Doctor of Philosophy Department of Chemical and Biological Engineering 2010 i This thesis entitled: Improving Palladium Catalysis with Self-Assembled Monolayers written by Stephen T. Marshall has been approved for the Department of Chemical and Biological Engineering J. William Medlin John L. Falconer Date The final copy of this thesis has been examined by the signatories, and we Find that both the content and the form meet acceptable presentation standards Of scholarly work in the above mentioned discipline. ii ABSTRACT Marshall, Stephen T. (Ph.D., Chemical and Biological Engineering) Improving Palladium Catalysis with Self-Assembled Monolayers Thesis directed by Associate Professor J. William Medlin Improving selectivity in catalytic systems is of primary interest to a number of fields. One means of achieving selectivity is through the use of promoters, or deposited materials that improve catalytic properties. Here, we present the modification of palladium surfaces with self- assembled monolayers (SAMs) formed from thiols. SAM coatings are employed in two systems: metal-insulator-semiconductor (MIS) sensors and supported palladium catalysts. On MIS sensors, modification with alkanethiol SAMs results in enhanced sensitivity to acetylene. Excellent selectivity for acetylene over ethylene is also observed. The functionalized sensors would serve as excellent acetylene detectors in a variety of applications including the detection of fault gases in transformers and detecting trace acetylene in ethylene production plants. -
N76-21508 Introduction
PHOTOCATALYTIC GENERATION OF HYDROGEN FROM WATER W.R. Bottoms and R.B. Miles Princeton University N76-21508 INTRODUCTION The dissociation of simple molecules provides a potentially efficient method of storing energy in a transportable form. Among the numerous chemical systems that have been proposed for energy conversion are the following: hv 2NOC1 ™ C\2 + 2NO hi> 2H20 ^ 2H2 + 02 2CO2 ^ 2CO + O2 Hydrogen offers the advantages of being a clean burning fuel, easily stored in gas, liquid, or hydride form, and efficiently transferable. It may be used as a fuel for a variety of energy conversion processes including fuel cells and rocket engines as well as conventional heat engines. In conjunction with a water dissociation process, it holds forth the promise of providing a closed cycle, photon powered, energy system where photon energy may be efficiently stored for future use and converted to thermal or electrical energy on demand. In this paper we will discuss a new concept which is designed to overcome the problems encountered when using photodissociation for the generation of hydrogen. Two fundamental problems limiting the efficiency of photodissociation of water are the separation of the photolysis products and the high energy photons necessary for the reaction. Although there has not been a great deal of work with metal-water systems, it has been shown that the dissociation energy of a large number of molecules is catalytically reduced when these molecules are in intimate contact with a surface of certain metals (ref. 1). The spontaneous dissociation of water has been demonstrated for Cu (ref. 2), Fe (ref. -
Frontiers at the Interface of Homogeneous and Heterogeneous Catalysis
FRONTIERS AT THE INTERFACE OF HOMOGENEOUS AND HETEROGENEOUS CATALYSIS Meeting of the Catalysis Science Program Chemical Sciences, Geosciences and Biosciences Division Office of Basic Energy Sciences U.S. Department of Energy Westin Annapolis Annapolis, Maryland June 30 – July 2, 2013 This document was produced under contract number DE-AC05-060R23100 between the U.S. Department of Energy and Oak Ridge Associated Universities. FOREWORD The 2013 Catalysis Science Program Meeting is sponsored by the Division of Chemical Sciences, Geosciences and Biosciences, Office of Basic Energy Sciences (BES), U.S. Department of Energy. It is being held on June 30 through July 2, 2013, at the Westin Annapolis Hotel, Annapolis, Maryland. The purposes of this meeting are to discuss the recent advances in the chemical, physical, and biological bases of catalysis science, to foster exchange of ideas and cooperation among participants, and to discuss the new challenges and opportunities recently emerging in energy technologies. Catalysis activities within BES emphasize fundamental research aimed at initially understanding and finally controlling the chemical conversion of natural and artificial feedstocks. The long-term goal of this research is to discover fundamental principles and produce ever more insightful approaches to predict structure-reactivity behavior. Such knowledge, integrated with advances in chemical and materials synthesis, in situ and operando analytical instrumentation, and chemical kinetics and quantum chemistry methods, will allow the control of chemical reactions along desired pathways. Ultimately, this new knowledge should impact the efficiency of conversion of natural resources into fuels, chemicals, materials, or other forms of energy, while minimizing the impact to the environment. This year’s meeting is focused on three topical areas: (i) the interface of homogeneous and heterogeneous catalysis, (ii) catalysis for biomass or solar energy conversion, and (iii) molecular catalysis, with an emphasis on organic synthesis. -
Scanned by Camscanner Scanned by Camscanner Scanned by Camscanner Scanned by Camscanner Scanned by Camscanner Scanned by Camscanner
Scanned by CamScanner Scanned by CamScanner Scanned by CamScanner Scanned by CamScanner Scanned by CamScanner Scanned by CamScanner Scanned by CamScanner Scanned by CamScanner Scanned by CamScanner Scanned by CamScanner Scanned by CamScanner Scanned by CamScanner Scanned by CamScanner Scanned by CamScanner Scanned by CamScanner Scanned by CamScanner Scanned by CamScanner Scanned by CamScanner Scanned by CamScanner Scanned by CamScanner Scanned by CamScanner Scanned by CamScanner Scanned by CamScanner Scanned by CamScanner Scanned by CamScanner Scanned by CamScanner Scanned by CamScanner Scanned by CamScanner Scanned by CamScanner Scanned by CamScanner Scanned by CamScanner Scanned by CamScanner Scanned by CamScanner Scanned by CamScanner Scanned by CamScanner Scanned by CamScanner Scanned by CamScanner Scanned by CamScanner Scanned by CamScanner Scanned by CamScanner Scanned by CamScanner Scanned by CamScanner Scanned by CamScanner Scanned by CamScanner Scanned by CamScanner Scanned by CamScanner Scanned by CamScanner Scanned by CamScanner Scanned by CamScanner Scanned by CamScanner Scanned by CamScanner Scanned by CamScanner Module 8 : Surface Chemistry Lecture 36 : Adsorption Objectives After studying this lecture, you will be able to Distinguish between physisorption and chemisorption. Distinguish between monolayer adsorption and multiplayer adsorption. Outline the main ingredients of the major isotherms. Distinguish quantitatively between the adsorption isotherms of Gibbs, Freundlich and Langmuir. Characterize a multiplayer adsorption through the BET isotherm. Determine the heat of reaction using an adsorption isotherm. Distinguish between the characteristics of bulk reactions and reactions at surfaces. Outline the mechanisms of unimolecular and bimolecular reactions at surfaces. List the applications of adsorption. 36.1 Adsorption The molecules at a surface of a material experience imbalanced forces of intermolecular interaction which contribute to the surface energy. -
IRVING LANGMUIR January 31, 1881-August 16, 1957
NATIONAL ACADEMY OF SCIENCES I R V I N G L ANGMUIR 1881—1957 A Biographical Memoir by C. GU Y S U I T S A N D M I L E S J . M ARTIN Any opinions expressed in this memoir are those of the author(s) and do not necessarily reflect the views of the National Academy of Sciences. Biographical Memoir COPYRIGHT 1974 NATIONAL ACADEMY OF SCIENCES WASHINGTON D.C. J). IRVING LANGMUIR January 31, 1881-August 16, 1957 BY C. GUY SUITS AND MILES J. MARTIN EW SCIENTISTS, in either university or industry, have made F as many, and as significant, contributions to scientific progress as did Dr. Irving Langmuir, the 1932 Nobel Prize winner in chemistry. It was on July 19, 1909, that Langmuir joined the General Electric Research Laboratory in which he was to become, first, assistant director and then associate direc- tor and which was to be the scene of his greatest achievements. One of Langmuir's first achievements was in the field of lighting. After Dr. William D. Coolidge, also of the Research Laboratory, developed the drawn tungsten-filament incandes- cent lamp, it fell to Langmuir to further develop an improved lamp—a gas-filled one instead of the vacuum type—and thereby make a great gain in lighting efficiency. The gas-filled lamp soon began driving arc lamps from the street lights, greatly increasing the use of electric lighting by increasing efficiency. With the lower cost of lighting came a large increase in the amount of light used, so that electric utility revenues from lighting were soon higher than ever before. -
Principles of Adsorption and Reaction on Solid Surfaces
PRINCIPLES OF ADSORPTION AND REACTION ON SOLID SURFACES Richard I. Masel Department of Chemical Engineering University of Illinois at Urbana-Champaign Urbana, Illinois A WILEY-INTERSCIENCE PUBLICATION JOHN WILEY & SONS, INC. New York • Chichester • Brisbane • Toronto • Singapore CONTENTS Preface xiii 1 Introduction 1 1.1 Historical Introduction 1 1.2 Overview of the Approach Taken in This Book 4 1.3 Techniques of Surface Analysis 5 2 The Structure of Solid Surfaces and Adsorbate Overlayers 15 2.1 Historical Introduction 15 2.2 Qualitative Description of Surface Structure 17 2.3 Quantitative Description of the Structure of Solids and Surfaces 20 2.3.1 Two-Dimensional Bravais Lattices 20 2.3.2 Extension to Slabs and Surfaces 26 2.3.3 Three-Dimensional Bravais Lattices 27 2.4 Miller Indices 33 2.5 The Structure of Solid Surfaces 37 2.5.1 Models of Ideal Single-Crystal Surfaces 37 2.5.2 The Stereographic Triangle 39 2.5.3 The Structure of Stepped Metal Surfaces 42 2.5.4 The Surface Structure of Semiconductor Crystals 46 2.6 Relaxations and Surface Reconstructions 48 2.6.1 Rare Gas Crystals 51 2.6.2 Metals 53 2.6.3 Elemental Semiconductor Surfaces 57 2.6.4 Ionic Crystal 59 2.6.5 Compound Semiconductors 62 2.7 Descriptive Notation for Surface Reconstructions 64 2.8 Site Notation 66 2.9 Roughening and Surface Melting 67 2.10 Solved Examples 68 2.11 Supplemental Material 89 2.11.1 Proof That There Can Only Be Two-, Three-, Four-, and Sixfold Axes in an Infinite Periodic Two- Dimensional Lattice 89 2.11.2 The Reciprocal Lattice 93 2.11.3 Somorjai -
12. Introduction to First-Principles Modeling of Catalysis at Surfaces
Introduction to first-principles modeling of catalysis at surfaces Bryan R. Goldsmith Fritz-Haber-Institut der Max-Planck-Gesellschaft Theory Department Catalysis is a multibillion-dollar industry and has a profound influence in everyday life Catalysts lower the activation barrier of a reaction without being consumed Images from presentation of Prof. Martin Wolf Early Nobel prizes for catalysis Paul Sabatier Wilhelm Ostwald “ for discoveries that confer the “for his method of hydrogenating "investigations into the fundamental greatest benefit on mankind” principles governing chemical organic compounds in the presence of equilibria and rates of reaction”, 1909 finely disintegrated metals", 1912 Irving Langmuir “for his discoveries and investigations Cyril Hinshelwood Fritz Haber, Berlin-Dahlem in surface chemistry”, 1932 "for researches into the mechanism “for the synthesis of ammonia of chemical reactions”, 1956 from its elements”, 1918 Some 21st-century Nobel prizes for catalysis What contributions can ab initio modeling provide to the catalysis field? Yves Chauvin, Lyon, France, 2005 Gerhard Ertl Fritz-Haber-Institut, Berlin “for his studies of chemical processes on solid surfaces”, 2007 CO + ½ O2 CO2 Pt Robert Grubbs, Richard Schrock Pasadena, CA, 2005 Boston, MA, 2005 → "for the development of the metathesis method in organic synthesis” Outline of this block course 1) Introduction to computational catalysis and first-principles modeling 2) Cluster models, slab models, ab initio thermodynamics and its applications 3) Transition state theory and potential energy surfaces --- 10 minute break 4) Computational screening of catalysts and the use of ‘descriptors’ 5) Topical Examples • Disintegration and redispersion of noble metal nanoparticles • Modeling isolated catalyst sites on amorphous supports Computational modeling can assist in understanding catalysis CO oxidation at RuO2 [1] K. -
Catalysis-Hub.Org, an Open Electronic Structure Database for Surface
www.nature.com/scientificdata OPEN Catalysis-Hub.org, an open ARTICLE electronic structure database for surface reactions Received: 1 February 2019 Kirsten T. Winther 1,2, Max J. Hofmann1,2, Jacob R. Boes1,2, Osman Mamun1,2, Accepted: 17 April 2019 Michal Bajdich1 & Thomas Bligaard1 Published: xx xx xxxx We present a new open repository for chemical reactions on catalytic surfaces, available at https:// www.catalysis-hub.org. The featured database for surface reactions contains more than 100,000 chemisorption and reaction energies obtained from electronic structure calculations, and is continuously being updated with new datasets. In addition to providing quantum-mechanical results for a broad range of reactions and surfaces from diferent publications, the database features a systematic, large-scale study of chemical adsorption and hydrogenation on bimetallic alloy surfaces. The database contains reaction specifc information, such as the surface composition and reaction energy for each reaction, as well as the surface geometries and calculational parameters, essential for data reproducibility. By providing direct access via the web-interface as well as a Python API, we seek to accelerate the discovery of catalytic materials for sustainable energy applications by enabling researchers to efciently use the data as a basis for new calculations and model generation. Introduction Electronic structure methods based on density functional theory (DFT) hold the promise to enable a deeper understanding of reaction mechanisms and reactivity trends for surface catalyzed chemical and electrochem- ical processes and eventually to accelerate discovery of new catalysts. As the access to large-scale supercom- puter resources continue to increase, the generated data from electronic structure calculations is also expected to increase1. -
Dynamic Processes on Gold-Based Catalysts Followed by Environmental Microscopies
catalysts Review Dynamic Processes on Gold-Based Catalysts Followed by Environmental Microscopies Eric Genty 1,*, Luc Jacobs 1, Thierry Visart de Bocarmé 1,2,* and Cédric Barroo 1,2 1 Chemical Physics of Materials and Catalysis, Université libre de Bruxelles, CP243, 1050 Brussels, Belgium; [email protected] (L.J.); [email protected] (C.B.) 2 Interdisciplinary Center for Nonlinear Phenomena and Complex Systems (CENOLI), Université libre de Bruxelles, 1050 Brussels, Belgium * Correspondence: [email protected] (E.G.); [email protected] (T.V.d.B.); Tel.: +32-2-650-5711 (E.G.); +32-2-650-5724 (T.V.d.B.) Academic Editors: Leonarda F. Liotta and Salvatore Scirè Received: 30 March 2017; Accepted: 20 April 2017; Published: 1 May 2017 Abstract: Since the early discovery of the catalytic activity of gold at low temperature, there has been a growing interest in Au and Au-based catalysis for a new class of applications. The complexity of the catalysts currently used ranges from single crystal to 3D structured materials. To improve the efficiency of such catalysts, a better understanding of the catalytic process is required, from both the kinetic and material viewpoints. The understanding of such processes can be achieved using environmental imaging techniques allowing the observation of catalytic processes under reaction conditions, so as to study the systems in conditions as close as possible to industrial conditions. This review focuses on the description of catalytic processes occurring on Au-based catalysts with selected in situ imaging techniques, i.e., PEEM/LEEM, FIM/FEM and E-TEM, allowing a wide range of pressure and material complexity to be covered. -
Principles of Adsorption and Reaction on Solid Surfaces
PRINCIPLES OF ADSORPTION AND REACTION ON SOLID SURFACES Richard I. Masel Department of Chemical Engineering University of Illinois at Urbana-Champaign Urbana, Illinois A WILEY-INTERSCIENCE PUBLICATION JOHN WILEY & SONS, INC. New York • Chichester • Brisbane • Toronto • Singapore CONTENTS Preface xiü 1 Introduction 1 1.1 Historical Introduction 1 1.2 Overview of die Approach Taken in This Book 4 1.3 Techniques of Surface Analysis 5 2 The Structure of Solid Surfaces and Adsorbate Overlayers 15 2.1 Historical Introduction 15 2.2 Qualitative Description of Surface Structure 17 2.3 Quantitative Description of the Structure of Solids and Surfaces 20 2.3.1 Two-Dimensional Bravais Lattices 20 2.3.2 Extension to Slabs and Surfaces 26 2.3.3 Three-Dimensional Bravais Lattices 27 2.4 Miller Indices 33 2.5 The Structure of Solid Surfaces 37 2.5.1 Models of Ideal Single-Crystal Surfaces 37 2.5.2 The Stereographic Triangle 39 2.5.3 The Structure of Stepped Metal Surfaces 42 2.5.4 The Surface Structure of Semiconductor Crystals 46 2.6 Relaxations and Surface Reconstructions 48 2.6.1 Rare Gas Crystals 51 2.6.2 Metals 53 2.6.3 Elemental Semiconductor Surfaces 57 2.6.4 Ionic Crystal 59 2.6.5 Compound Semiconductors 62 2.7 Descriptive Notation for Surface Reconstructions 64 2.8 Site Notation 66 2.9 Roughening and Surface Melting 67 2.10 Solved Examples 68 2.11 Supplemental Material 89 2.11.1 Proof That There Can Only Be Two-, Three-, Four-, and Sixfold Axes in an Infinite Periodic Two- Dimensional Lattice 89 2.11.2 The Reciprocal Lattice 93 2.11.3 Somorjai -
Ethanol Reactions Over the Surfaces of Noble Metal/Cerium Oxide Catalysts
DOI: 10.1595/147106704X1603 Ethanol Reactions over the Surfaces of Noble Metal/Cerium Oxide Catalysts By H. Idriss The University of Auckland, Department of Chemistry, Auckland, New Zealand; E-mail: [email protected] This review focuses on the reactions of ethanol on the surfaces of platinum, palladium, rhodium and gold supported on ceria (of size 1020 nm). The bimetallic compounds: Pt-Rh, Rh-Au, Rh-Pd, and Pt-Pd were also investigated. Initially this work was aimed at understanding the roles of the different components of automobile catalytic converters on the reactions of ethanol, which is used as a fuel additive. Some of the catalysts that showed high activity for ethanol oxidation were also investigated for hydrogen production. The addition of any of the above metals to CeO2 was found to suppress the oxidation of ethanol to acetates at room temperature, as there are fewer surface oxygen atoms available to oxidise the ethanol (the remaining oxygen atoms did not produce efficient oxidation). Ethanol dehydrogenation to acetaldehyde was facilitated by the presence of Pt or Pd; at higher temperatures the acetaldehyde condensed to other organic compounds, such as crotonaldehyde. By contrast, in the presence of Rh only traces of acetaldehyde or other organic compounds were seen on the surface, and detectable amounts of CO were found upon ethanol adsorption at room temperature. This indicates the powerful nature of Rh in breaking the carbon-carbon bond in ethanol. The effects of prior reduction were also investigated and clear differences were seen: for example, a shift in reaction selectivity is observed for the bimetallic Rh-containing catalysts.