Copyright by Alexander Gary Fay 2014 the Dissertation Committee for Alexander Gary Fay Certifies That This Is the Approved Version of the Following Dissertation
Total Page:16
File Type:pdf, Size:1020Kb
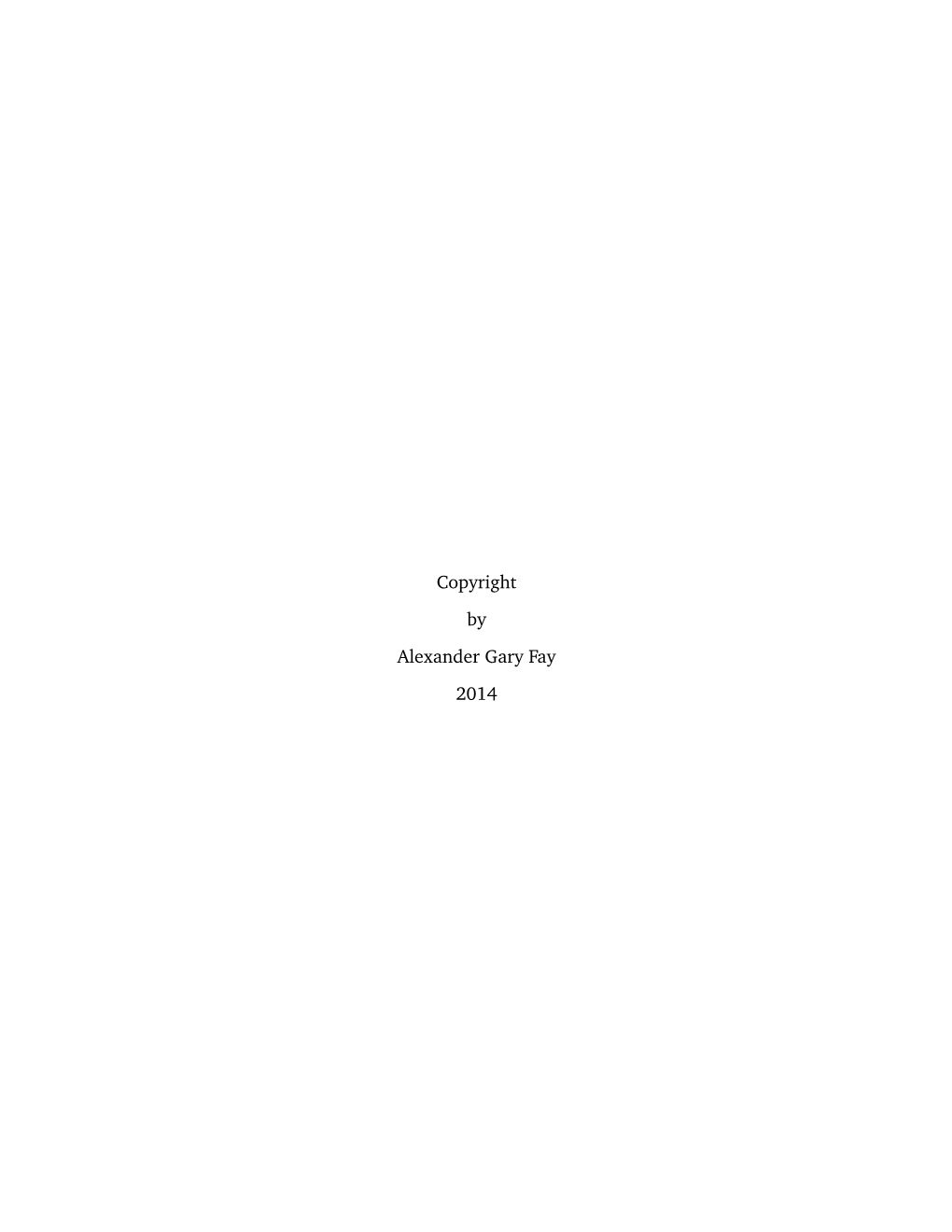
Load more
Recommended publications
-
12 Natural Isotopes of Elements Other Than H, C, O
12 NATURAL ISOTOPES OF ELEMENTS OTHER THAN H, C, O In this chapter we are dealing with the less common applications of natural isotopes. Our discussions will be restricted to their origin and isotopic abundances and the main characteristics. Only brief indications are given about possible applications. More details are presented in the other volumes of this series. A few isotopes are mentioned only briefly, as they are of little relevance to water studies. Based on their half-life, the isotopes concerned can be subdivided: 1) stable isotopes of some elements (He, Li, B, N, S, Cl), of which the abundance variations point to certain geochemical and hydrogeological processes, and which can be applied as tracers in the hydrological systems, 2) radioactive isotopes with half-lives exceeding the age of the universe (232Th, 235U, 238U), 3) radioactive isotopes with shorter half-lives, mainly daughter nuclides of the previous catagory of isotopes, 4) radioactive isotopes with shorter half-lives that are of cosmogenic origin, i.e. that are being produced in the atmosphere by interactions of cosmic radiation particles with atmospheric molecules (7Be, 10Be, 26Al, 32Si, 36Cl, 36Ar, 39Ar, 81Kr, 85Kr, 129I) (Lal and Peters, 1967). The isotopes can also be distinguished by their chemical characteristics: 1) the isotopes of noble gases (He, Ar, Kr) play an important role, because of their solubility in water and because of their chemically inert and thus conservative character. Table 12.1 gives the solubility values in water (data from Benson and Krause, 1976); the table also contains the atmospheric concentrations (Andrews, 1992: error in his Eq.4, where Ti/(T1) should read (Ti/T)1); 2) another category consists of the isotopes of elements that are only slightly soluble and have very low concentrations in water under moderate conditions (Be, Al). -
A Double-Spike Method for K∓Ar Measurement: a Technique for High
Available online at www.sciencedirect.com Geochimica et Cosmochimica Acta 110 (2013) 1–12 www.elsevier.com/locate/gca A double-spike method for K–Ar measurement: A technique for high precision in situ dating on Mars and other planetary surfaces K.A. Farley a,⇑, J.A. Hurowitz b, P.D. Asimow a, N.S. Jacobson c, J.A. Cartwright a a Division of Geological and Planetary Sciences, Caltech, Pasadena, CA 91125, USA b Jet Propulsion Laboratory, Caltech, Pasadena, CA 91109, USA c NASA Glenn Research Center, Cleveland, OH 44135, USA Received 4 December 2012; accepted in revised form 5 February 2013; Available online 16 February 2013 Abstract A new method for K–Ar dating using a double isotope dilution technique is proposed and demonstrated. The method is designed to eliminate known difficulties facing in situ dating on planetary surfaces, especially instrument complexity and power availability. It may also have applicability in some terrestrial dating applications. Key to the method is the use of a solid tracer spike enriched in both 39Ar and 41K. When mixed with lithium borate flux in a Knudsen effusion cell, this tracer spike and a sample to be dated can be successfully fused and degassed of Ar at <1000 °C. The evolved 40Ar*/39Ar ratio can be measured to high precision using noble gas mass spectrometry. After argon measurement the sample melt is heated to a slightly higher temperature (1030 °C) to volatilize potassium, and the evolved 39K/41K ratio measured by Knudsen effusion mass spectrometry. Combined with the known composition of the tracer spike, these two ratios define the K–Ar age using a single sample aliquot and without the need for extreme temperature or a mass determination. -
Dating of Groundwater with Atom Trap Trace Analysis of Ar
blabla Dating of groundwater with Atom Trap Trace Analysis of 39Ar Florian Ritterbusch Issued 2013 Dissertation submitted to the Combined Faculties of the Natural Sciences and Mathematics of the Ruperto-Carola-University of Heidelberg, Germany for the degree of Doctor of Natural Sciences Put forward by Florian Ritterbusch born in: Bielefeld, Germany Oral examination: February 05, 2014 blabla Dating of groundwater with Atom Trap Trace Analysis of 39Ar Referees: Prof. Dr. Markus K. Oberthaler Prof. Dr. Norbert Frank Zusammenfassung Das Radioisotop 39Ar, mit einer Halbwerszeit von 269 a, kann zur Datierung von Wasser und Eis im Zeitbereich von 50–1000 a, in dem keine andere verlässliche Datierungsmethode besteht, verwendet werden. Aufgrund seiner extrem kleinen Häufigkeit von nur 8:23 10−16, kann 39Ar derzeit nur durch Low-Level Counting · (LLC) in dem Untergrundlabor der Universität Bern gemessen werden. Atom Trap Trace Analysis (ATTA) ist eine atom-optische Methode die zur Anal- yse seltener Krypton-Isotope entwickelt wurde. Im Rahmen dieser Arbeit wurde eine ATTA-Apparatur zur Einzelatomdetektion von 39Ar realisiert und angewendet auf die Datierung von Grundwasserproben. Die 39Ar-Kontamination in der Apparatur, welche von der Optimierung mit an- gereicherten Proben stammt, konnte mit Hilfe einer Probe bestimmt werden, deren Konzentration mit LLC auf < 5 % modern gemessen wurde. Wird die resultierende Untergrundzählrate 0:38(18) atoms/h zur Korrektur verwendet, so ergibt sich eine atmosphärische 39Ar-Zählrate von 3:22(21) atoms/h. Basierend auf kontrollierten Mischungen von atmosphärischem Argon und einer Probe mit bekannter 39Ar-Konzentration von 9(5) % modern konnte die Anwendung der vorgestellten Methode auf die Datierung von Wasser validiert werden. -
Oqnf-9203164—1 De92 016800 Exotic Nuclear Shapes And
OQNF-9203164—1 DE92 016800 EXOTIC NUCLEAR SHAPES AND CONFIGURATIONS THAT CAN BE STUDIED AT HIGH SPIN USING RADIOACTIVE ION BEAMS J. D. Garrett Physics Division, Oak Ridge National Laboratory, Oak Ridge, Tennessee 37831-6371, USA ABSTRACT The variety of new research possibilities afforded by the culmination of the two frontier areas of nuclear structure: high spin and studies far from nuclear stability (utilizing intense radioactive ion beams) are discussed. Topics presented include: new regions of exotic nuclear shape (e.g. superdeformation, hyperdeformation, and reflection-asymmetric shapes); the population of and consequences of populating exotic nuclear configurations; and complete spectroscopy (i.e. the overlap of state of the art low- and high-spin studies in the same nucleus). Likewise, the various beams needed for proton- and neutron-rich high spin studies also are discussed. DISCLAIMER This report was prepared as an account of work sponsored by an agency of the United Slates Government. Neither the United States Government nor any agency thereof, nor any of their employees, makes any warranty, express or implied, or assumes any legal liability or responsi- bility for the accuracy, completeness, or usefulness of any information, apparatus, product, or process disclosed, or represents that its use would not infringe privately owned rights. Refer- ence herein to any specific commercial product, process, or service by trade name, trademark, manufacturer, or otherwise does not necessarily constitute or imply its endorsement, rccom- mendaiiun, or favoring by the United Stales Government or any agency thereof. The views and opinions of authors expressed herein do not necessarily slate or reflect those of the United Stiles Government or any agency thereof. -
Compelling Research Opportunities Using Isotopes (NSAC Report)
Final Report One of Two 2008 Charges to NSAC on the National Isotopes Production and Applications Program Compelling Research Opportunities using Isotopes NSAC Isotopes Subcommittee April 23, 2009 The Cover: The discovery of isotopes is less than 100 years old, today we are aware of about 250 stable isotopes of the 90 naturally occurring elements. The number of natural and artificial radioactive isotopes exceeds 3200, already, and this number keeps growing every year. "Isotope" originally meant elements that are chemically identical and non‐separable by chemical methods. Now isotopes can be separated by a number of methods such as distillation or electromagnetic separation.The strong colors and the small deviations from one to the other indicate the small differences between isotopes that yield their completely different properties in therapy, in nuclear science, and a broad range of other applications. The surrounding red, white and blue theme highlights the broad national impact of the US National Isotope Production and Application program. 1 Table of Contents Executive Summary Chapter 1 Introduction and Background Chapter 2 Landscape of Isotope Production in the US as of 2009 Chapter 3 Research Opportunities with Isotopes in Biology, Medicine, and Pharmaceuticals Chapter 4 Research Opportunities with Isotopes in Physical Sciences and Engineering Chapter 5 Research Opportunities with Isotopes in National Security Chapter 6 Recommendations for the most compelling research opportunities with Isotopes across the various disciplines Appendix 1 DOE Office of Nuclear Physics Charge to Nuclear Science Advisory Committee (NSAC) Appendix 2 Membership of the NSAC Isotopes Subcommittee (NSACI) Appendix 3 Agendas of NSACI Meetings I, II, and III Appendix 4 List of Agencies Contacted by NSACI Appendix 5 List of Professional Societies Contacted by NSACI 2 Executive Summary Isotopes are vital to the science and technology base of the US economy. -
Explorations of Magnetic Properties of Noble Gases: the Past, Present, and Future
magnetochemistry Review Explorations of Magnetic Properties of Noble Gases: The Past, Present, and Future Włodzimierz Makulski Faculty of Chemistry, University of Warsaw, 02-093 Warsaw, Poland; [email protected]; Tel.: +48-22-55-26-346 Received: 23 October 2020; Accepted: 19 November 2020; Published: 23 November 2020 Abstract: In recent years, we have seen spectacular growth in the experimental and theoretical investigations of magnetic properties of small subatomic particles: electrons, positrons, muons, and neutrinos. However, conventional methods for establishing these properties for atomic nuclei are also in progress, due to new, more sophisticated theoretical achievements and experimental results performed using modern spectroscopic devices. In this review, a brief outline of the history of experiments with nuclear magnetic moments in magnetic fields of noble gases is provided. In particular, nuclear magnetic resonance (NMR) and atomic beam magnetic resonance (ABMR) measurements are included in this text. Various aspects of NMR methodology performed in the gas phase are discussed in detail. The basic achievements of this research are reviewed, and the main features of the methods for the noble gas isotopes: 3He, 21Ne, 83Kr, 129Xe, and 131Xe are clarified. A comprehensive description of short lived isotopes of argon (Ar) and radon (Rn) measurements is included. Remarks on the theoretical calculations and future experimental intentions of nuclear magnetic moments of noble gases are also provided. Keywords: nuclear magnetic dipole moment; magnetic shielding constants; noble gases; NMR spectroscopy 1. Introduction The seven chemical elements known as noble (or rare gases) belong to the VIIIa (or 18th) group of the periodic table. These are: helium (2He), neon (10Ne), argon (18Ar), krypton (36Kr), xenon (54Xe), radon (86Rn), and oganesson (118Og). -
Paper 1 Chemistry
Write your name here Surname Other names Centre Number Candidate Number Pearson Edexcel Level 1/Level 2 GCSE (9-1) Combined Science Paper 3: Chemistry 1 Foundation Tier Thursday 17 May 2018 – Morning Paper Reference Time: 1 hour 10 minutes 1SC0/1CF You must have: Total Marks Calculator, ruler Instructions • Use black ink or ball-point pen. • Fill in the boxes at the top of this page with your name, centre number and candidate number. • Answer all questions. • Answer the questions in the spaces provided – there may be more space than you need. • Calculators may be used. • Any diagrams may NOT be accurately drawn, unless otherwise indicated. • You must show all your working out with your answer clearly identified at the end of your solution. Information • The total mark for this paper is 60 • The marks for each question are shown in brackets – use this as a guide as to how much time to spend on each question. • In questions marked with an asterisk (*), marks will be awarded for your ability to structure your answer logically showing how the points that you make are related or follow on from each other where appropriate. • A periodic table is printed on the back cover of this paper. Advice • Read each question carefully before you start to answer it. • Try to answer every question. • Check your answers if you have time at the end. Turn over P59176RA ©2018 Pearson Education Ltd. *P59176RA0120* 1/1/1/ THIS AREA WRITE IN DO NOT Answer ALL questions. Write your answers in the spaces provided. Some questions must be answered with a cross in a box . -
Diffusion of Krypton in Cesium and Rubidium Iodide; a Comparison of Results Obtained Following Reactor Irradiation and Ion Bombardment
Diffusion of Krypton in Cesium and Rubidium Iodide; A Comparison of Results Obtained Following Reactor Irradiation and Ion Bombardment Hj. MATZKE * and F. SPRINGER High Temperature Chemistry Group, CCR EURATOM, Ispra (Va), Italy (Z. Naturforsch. 25 a, 252—256 [1970] ; received 29 October 1969) The present study shows that under suitably chosen conditions, the same results of rare gas diffusion coefficients can be obtained in samples which are reactor irradiated to produce a homo- geneous concentration of rare gas, and in samples that are labeled with rare gas by controlled ion bombardment. In the present study, the diffusion of krypton in Rbl and Csl was found to follow the empirical rule of yielding activation enthalpies in the range (1.4±0.2) x 10~3 Tm eV, with Tm = melting point in °K. Trapping of rare gases (gas-gas or gas-damage interactions) was observed at high gas concentration. Rare gas diffusion constants are usually deter- and used without waiting times between labeling mined by measuring the integral amount of gas that and release experiment whereas reactor irradiated diffuses out of the specimen, i. e. the amount of gas samples are frequently highly radioactive and wait- that is released from the solid. In the early investi- ing times are necessary if all or part of the gas gations, reactor irradiation was used to produce the atoms are not produced directly but originate by rare gas atoms in the solid by a suitable nuclear radioactive decay of a mother substance; the effect reaction [fission, (n,y), (n,oc), (n,p) etc.]. -
Atoms: the Building Blocks of Matter
CHAPTER 3 Atoms: The Building Blocks of Matter An atom is the smallest particle of an element that retains the chemical properties of that element. Copyright © by Holt, Rinehart and Winston. All rights reserved. The Atom: From SECTION 3-1 Philosophical Idea to OBJECTIVES Explain the law of conservation of mass, the Scientific Theory law of definite proportions, and the law of multiple proportions. Summarize the five essential W hen you crush a lump of sugar, you can see that it is made up of points of Dalton’s atomic many smaller particles of sugar.You may grind these particles into a very theory. fine powder, but each tiny piece is still sugar. Now suppose you dissolve the sugar in water.The tiny particles seem to disappear completely. Even Explain the relationship if you look at the sugar-water solution through a powerful microscope, between Dalton’s atomic you cannot see any sugar particles. Yet if you were to taste the solution, theory and the law of conser- you’d know that the sugar is still there. Observations like these led early vation of mass, the law of philosophers to ponder the fundamental nature of matter. Is it continu- definite proportions, and the ous and infinitely divisible, or is it divisible only until a basic, invisible law of multiple proportions. particle that cannot be divided further is reached? The particle theory of matter was supported as early as 400 B.C.by certain Greek thinkers, such as Democritus. He called nature’s basic par- ticle an atom, based on the Greek word meaning “indivisible.” Aristotle was part of the generation that succeeded Democritus. -
Imploveients in METHODS of EXTRACTION, PURIFICATION, A1D 1ASUREIENT of EADIO
IMPLOVEiENTS IN METHODS OF EXTRACTION, PURIFICATION, A1D 1ASUREIENT OF EADIO GENIC ARGON IN MINEAiLS by LAWRENCE STRICKLLND S.B., Massachusetts Institute of Technology (1952) SUBMITTED IN PARTIAL FULFILLIENT OF THE REQUIREIENTS FOR THE DEGREE OF DOCTOR OF PHILOSOPHY at the MASSACHUSETTS INSTITUTE OF TECHNOLOGY June, 1956 Signature of Author. ...... *0.-.. *a *.. , .... Department 4f Geology and Geophysics / X, Of .I Septe;nber p3, 1955 Certified Thepc SupgrvAsor Accepted by.\......... 4.................. .... Chairman, Departmental Committee on Gradua Students -Ming== A CINOWLEDGEMvENTS The author is indebted to the many people who helped in the completion of this research. He wishes to thank Dr. Leonard Herzog, who was always available for consultation when problems arose involving mass spectrometry and who was an invaluable help in the early stages of this re- search. Professor Patrick Hurley, who suggested the author undertake this research and who was always willing to take time from his busy schedule to help. He was a source of inspiration whenever forward progress was slow. The author will remember his association with Professor Hurley for many years. Mr. Milo Backus, whose companion- ship made the many hours spent on this research seem short. The typist, Joan Whitehouse, for her untiring efforts to complete this manuscriot in a tight schedule. His wife, Shirley, without her unlimited confidence in the author, and unselfish devotion, the successful completion of this research would have been impossible. The entire staff of the Geology Department. The research presented in this thesis was a part of a program supported by the Atomic Energy Commission urAer Contract AT(30-1)-1381. -
The Low-Radioactivity Underground Argon Workshop a Workshop Synopsis Thomas Alexander1, Henning O
The Low-Radioactivity Underground Argon Workshop A workshop synopsis Thomas Alexander1, Henning O. Back1,*, Walter Bonivento2, Mark Boulay3, Philippe Collon4, Zhongyi Feng5, Michael Foxe1, Pablo García Abia6, Pietro Giampa7, Christopher Jackson1, Christine Johnson1, Emily Mace1, Peter Mueller8, László Palcsu9, Walter Pettus10, Roland Purtschert11, Andrew Renshaw12, Richard Saldanha1, Kate Scholberg13, Marino Simeone14, Ondřej Šrámek15, Rex Tayloe16, Ward TeGrotenhuis1, Signe White1, Richard Williams1 1. Pacific Northwest National Laboratory, Richland, Washington 99352, USA 2. INFN Cagliari, Cagliari 09042, Italy 3. Carleton University, Ottawa, Ontario K1S 5B6, Canada 4. University of Notre Dame, Notre Dame, Indiana 46556, USA 5. Kirchhoff-Institut für Physik, Universität Heidelberg, 69120 Heidelberg, Germany 6. CIEMAT, Centro de Investigaciones Energéticas, Medioambientales y Tecnológicas, Madrid 28040, Spain 7. TRIUMF, Vancouver, British Columbia V6T 2A3, Canada 8. Argonne National Laboratory, Lemont, Illinois 60439, USA 9. Institute for Nuclear Research, Hungarian Academy of Sciences, H-4001 Debrecen, Hungary 10. University of Washington, Seattle, Washington 98195, USA 11. University of Bern, 3012 Bern, Switzerland 12. University of Houston, Houston, Texas 77204, USA 13. Duke University, Durham, North Carolina 27708, USA 14. Università degli Studi di Napoli “Federico II”, Napoli 80125, Italy 15. Department of Geophysics, Faculty of Mathematics and Physics, Charles University, 180000 Prague, Czech Republic 16. Indiana University, Bloomington, -
By August 1964
THE MARINE GEOCHEMISTRY OF ARGON By David Frank Bolka Submitted in Partial Fulfillment of the Requirements for the Degrees of Bachelor of Science and Master of Science at the MASSACHUSETTS INSTITUTE OF TECHNOLOGY August 1964 Signature of Author, Department of Geo_lo d Geophysics, August 24, 1964 Certified by - - - - - - -- - Thesis Supervisor Accepted by Cha man. Departmental Committee on Graduate Students THE MARINE GEOCIHEMSTRY OF ARGON By David Frank Bolka Submitted to the Department of Geology and eophysics on August 24, 1904, in partial fulfillment atof the requirements for the degrees ot Bachelor of Science and Master ofat Science. ABSTRACT An extensive literature search has been made with the attempt to collect all references to the solubility o arg n distilled water, aqueous salt solutions and sea water except for untranslated Russian literature. The bibliography is considered to be a significant collection of work up to the present time coneerning not only the solubtity of argon, bu the sources and the distribution o argon in the osmos thospremoshospheospheres biosphere and bydrosphere. The prperties and processes of formation of argon isotopes are discussed and the decay at potassium-40 to -argon40to treated at length with calculations at the amounts of argon-40 Introduced into the oosans by this process. These amounts are shown to be negli- gible for any conceivable residence time of deep water. The solahity of argon in distilled water and in sea water $s discussed with references to original papers of all investigators who have been cncerned with this problem. The effect at hydrostatic pressure a the solaubilty o argon is discussed theoretically and figures are given to show that the equilibrium model at the argon cycle in the sea is incorrect.