Holocrine Secretion and Kino Flow in Angiosperms
Total Page:16
File Type:pdf, Size:1020Kb
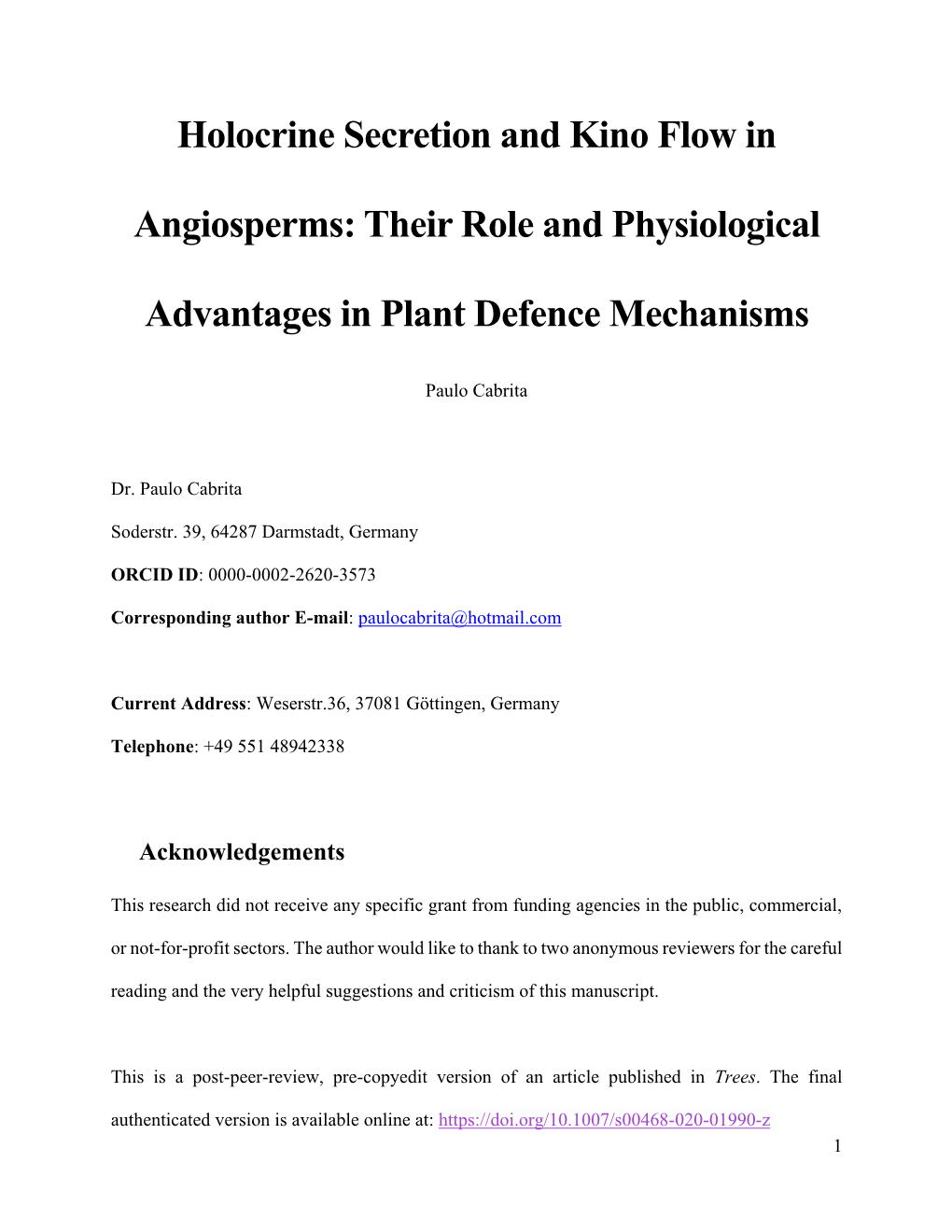
Load more
Recommended publications
-
Final Recovery Plan Southwestern Willow Flycatcher (Empidonax Traillii Extimus)
Final Recovery Plan Southwestern Willow Flycatcher (Empidonax traillii extimus) August 2002 Prepared By Southwestern Willow Flycatcher Recovery Team Technical Subgroup For Region 2 U.S. Fish and Wildlife Service Albuquerque, New Mexico 87103 Approved: Date: Disclaimer Recovery Plans delineate reasonable actions that are believed to be required to recover and/or protect listed species. Plans are published by the U.S. Fish and Wildlife Service, sometimes prepared with the assistance of recovery teams, contractors, State agencies, and others. Objectives will be attained and any necessary funds made available subject to budgetary and other constraints affecting the parties involved, as well as the need to address other priorities. Recovery plans do not necessarily represent the views nor the official positions or approval of any individuals or agencies involved in the plan formulation, other than the U.S. Fish and Wildlife Service. They represent the official position of the U.S. Fish and Wildlife Service only after they have been signed by the Regional Director or Director as approved. Approved Recovery plans are subject to modification as dictated by new findings, changes in species status, and the completion of recovery tasks. Some of the techniques outlined for recovery efforts in this plan are completely new regarding this subspecies. Therefore, the cost and time estimates are approximations. Citations This document should be cited as follows: U.S. Fish and Wildlife Service. 2002. Southwestern Willow Flycatcher Recovery Plan. Albuquerque, New Mexico. i-ix + 210 pp., Appendices A-O Additional copies may be purchased from: Fish and Wildlife Service Reference Service 5430 Governor Lane, Suite 110 Bethesda, Maryland 20814 301/492-6403 or 1-800-582-3421 i This Recovery Plan was prepared by the Southwestern Willow Flycatcher Recovery Team, Technical Subgroup: Deborah M. -
U·M·I University Microfilms International a 8Ell & Howell Information Company 300 North Zeeb Road
Patterns of homoplasy in North American Astragalus L. (Fabaceae). Item Type text; Dissertation-Reproduction (electronic) Authors Sanderson, Michael John. Publisher The University of Arizona. Rights Copyright © is held by the author. Digital access to this material is made possible by the University Libraries, University of Arizona. Further transmission, reproduction or presentation (such as public display or performance) of protected items is prohibited except with permission of the author. Download date 10/10/2021 18:39:52 Link to Item http://hdl.handle.net/10150/184764 INFORMATION TO USERS The most advanced technology has been used to photo graph and reproduce this manuscript from the microfilm master. UMI films the text directly from the original or copy submitted. Thus, some thesis and dissertation copies are in typewriter face, while others may be from any type of computer printer. The quality of this reproduction is dependent upon the quality of the copy submitted. Broken or indistinct print, colored or poor quality illustrations and photographs, print bleedthrough, substandard margins, and improper alignment can adversely affect reproduction. In the unlikely event that the author did not send UIVn a complete manuscript and there are missing pages, these will be noted. Also, if unauthorized copyright material had to be removed, a note will indicate the deletion. Oversize materials (e.g., maps, drawings, charts) are re produced by sectioning the original, beginning at the upper left-hand corner and continuing from left to right in equal sections with small overlaps. Each original is also photographed in one exposure and is included in reduced form at the back of the book. -
Planning Commission
Revised Arborist Report 300 Montague Expressway Milpitas, CA Prepared for: Trumark Companies 4185 Blackhawk Plaza Circle, Suite 200 Danville, CA 94506 Prepared by: HortScience, Inc. 2150 Rheem Dr., Suite A Pleasanton, CA 94588 October, 2011 Revised Arborist Report 300 Montague Expressway Milpitas Table of Contents Page Introduction and Overview 1 Survey Methods 1 Description of Trees 2 Suitability for Preservation 4 Evaluation of Impacts 5 Tree Preservation Guidelines 7 List of Tables Table 1. Tree condition and frequency of occurrence 3 Table 2. Suitability for Preservation 5 Table 3. Recommendations for preservation 6 Attachments Tree Assessment Form Tree Assessment Map Revised Arborist Report, Trumark Companies HortScience, Inc. 300 Montague Expressway, Milpitas Page 1 Introduction and Overview Trumark Companies are proposing to redevelop the property located at 300 Montague Expressway, in Milpitas. The site is triangular, bordered by Montague Expressway to the west and Trade Zone Blvd. to the south. Currently, the site is an office complex, with a vegetated berm along the western and southern boundaries. The three buildings are centrally located with peripheral parking and landscaping throughout. The plan proposes to construct 92 attached town homes and 42 single-family homes, for a total of 134 homes. HortScience, Inc. was asked to prepare an Arborist Report for the site. This report provides the following information: 1. An evaluation of the health and structural condition of all trees growing within and adjacent to the project area based on a visual inspection of external conditions. 2. An assessment of the impacts of constructing the proposed project on the trees. 3. Guidelines for tree preservation during the design, construction and maintenance phases of development. -
Evolutionary Relationships in Eucalyptus Sens. Lat. – a Synopsis
Euclid - Online edition Evolutionary relationships in Eucalyptus sens. lat. – a synopsis This article complements the introductory essay about eucalypts included in the "Learn about Eucalypts" section. Its aim is to provide an up-to-date account of the outcomes of research derived from different groups during the past 5 years relating to relationships within Eucalyptus s.s. As such it includes only those publications and hypotheses relating to higher level relationships of major groupings within the eucalypts. Some of the research reported below also provides insights into biogeographic relationships of the eucalypt group – in large part these are not the focus of this article and are not discussed in detail. Introduction The first comprehensive classification of the eucalypts was published by Blakely in 1934, in which he treated more than 600 taxa, building on earlier work of Maiden and Mueller. Blakely's classification remained the critical reference for Eucalyptus taxonomists for the next 37 years when a new but informal classification was published by Pryor and Johnson (1971). In this work the authors divided the genus into seven subgenera, and although of an informal nature, presented a system of great advance on Blakely's treatment. The small genus Angophora was retained. The next 20 years saw much debate about the naturalness of Eucalyptus and whether other genera should be recognized (e.g., Johnson 1987). Based on morphological data, Hill and Johnson in 1995 proposed a split in the genus and recognition of the genus Corymbia. This new genus of c. 113 species, comprised the ghost gums and the bloodwoods, and Hill and Johnson concluded that Corymbia is the sister group to Angophora, with the synapomorphy of the distinctive cap cells on bristle glands (Ladiges 1984) being unambiguous. -
Genera in Myrtaceae Family
Genera in Myrtaceae Family Genera in Myrtaceae Ref: http://data.kew.org/vpfg1992/vascplnt.html R. K. Brummitt 1992. Vascular Plant Families and Genera, Royal Botanic Gardens, Kew REF: Australian – APC http://www.anbg.gov.au/chah/apc/index.html & APNI http://www.anbg.gov.au/cgi-bin/apni Some of these genera are not native but naturalised Tasmanian taxa can be found at the Census: http://tmag.tas.gov.au/index.aspx?base=1273 Future reference: http://tmag.tas.gov.au/floratasmania [Myrtaceae is being edited at mo] Acca O.Berg Euryomyrtus Schaur Osbornia F.Muell. Accara Landrum Feijoa O.Berg Paragonis J.R.Wheeler & N.G.Marchant Acmena DC. [= Syzigium] Gomidesia O.Berg Paramyrciaria Kausel Acmenosperma Kausel [= Syzigium] Gossia N.Snow & Guymer Pericalymma (Endl.) Endl. Actinodium Schauer Heteropyxis Harv. Petraeomyrtus Craven Agonis (DC.) Sweet Hexachlamys O.Berg Phymatocarpus F.Muell. Allosyncarpia S.T.Blake Homalocalyx F.Muell. Pileanthus Labill. Amomyrtella Kausel Homalospermum Schauer Pilidiostigma Burret Amomyrtus (Burret) D.Legrand & Kausel [=Leptospermum] Piliocalyx Brongn. & Gris Angasomyrtus Trudgen & Keighery Homoranthus A.Cunn. ex Schauer Pimenta Lindl. Angophora Cav. Hottea Urb. Pleurocalyptus Brongn. & Gris Archirhodomyrtus (Nied.) Burret Hypocalymma (Endl.) Endl. Plinia L. Arillastrum Pancher ex Baill. Kania Schltr. Pseudanamomis Kausel Astartea DC. Kardomia Peter G. Wilson Psidium L. [naturalised] Asteromyrtus Schauer Kjellbergiodendron Burret Psiloxylon Thouars ex Tul. Austromyrtus (Nied.) Burret Kunzea Rchb. Purpureostemon Gugerli Babingtonia Lindl. Lamarchea Gaudich. Regelia Schauer Backhousia Hook. & Harv. Legrandia Kausel Rhodamnia Jack Baeckea L. Lenwebia N.Snow & ZGuymer Rhodomyrtus (DC.) Rchb. Balaustion Hook. Leptospermum J.R.Forst. & G.Forst. Rinzia Schauer Barongia Peter G.Wilson & B.Hyland Lindsayomyrtus B.Hyland & Steenis Ristantia Peter G.Wilson & J.T.Waterh. -
Dragon's Blood
Available online at www.sciencedirect.com Journal of Ethnopharmacology 115 (2008) 361–380 Review Dragon’s blood: Botany, chemistry and therapeutic uses Deepika Gupta a, Bruce Bleakley b, Rajinder K. Gupta a,∗ a University School of Biotechnology, GGS Indraprastha University, K. Gate, Delhi 110006, India b Department of Biology & Microbiology, South Dakota State University, Brookings, South Dakota 57007, USA Received 25 May 2007; received in revised form 10 October 2007; accepted 11 October 2007 Available online 22 October 2007 Abstract Dragon’s blood is one of the renowned traditional medicines used in different cultures of world. It has got several therapeutic uses: haemostatic, antidiarrhetic, antiulcer, antimicrobial, antiviral, wound healing, antitumor, anti-inflammatory, antioxidant, etc. Besides these medicinal applica- tions, it is used as a coloring material, varnish and also has got applications in folk magic. These red saps and resins are derived from a number of disparate taxa. Despite its wide uses, little research has been done to know about its true source, quality control and clinical applications. In this review, we have tried to overview different sources of Dragon’s blood, its source wise chemical constituents and therapeutic uses. As well as, a little attempt has been done to review the techniques used for its quality control and safety. © 2007 Elsevier Ireland Ltd. All rights reserved. Keywords: Dragon’s blood; Croton; Dracaena; Daemonorops; Pterocarpus; Therapeutic uses Contents 1. Introduction ........................................................................................................... -
Cafe-Kino-Menu-January-2019.Pdf
Cafe Kino is a not-for-profit workers’ cooperative, here to serve the local community. All of our food is vegan, our meals are lovingly handmade on site and most can be ordered to take-away. Please order at the counter with your table number. Burgers Snacks Let us know if you have any allergies, including gluten Our famous burgers are handmade by us from £2.40 intolerance, when you order your food. Freshly baked vegan sausage roll organic UK grown badger beans, carrots, beetroot, Slice of farinata (gf) – Italian chickpea flour tart £2.20 Breakfast served from 10am until 1pm oats, herbs and seeds. All served in a granary bap with our own Kino mayonnaise Pitta bread with homemade hummus £3.20 Full Kino – two vegan sausages, two hash browns, £9.00 Olives (gf) in homemade marinade £2.20 garlic mushrooms, roast tomato, baked beans, Classic Kino Burger – with tangy tomato relish, £5.80 seasonal greens and granary toast fresh leaves and tomato Crisps – varieties as stocked £1.60 Small Kino – vegan sausage, hash brown, garlic £7.00 Spicy Burger – with our homemade hot chilli £5.80 mushrooms, roast tomato, baked beans and sauce, relish, salsa, fresh leaves and tomato Sides (ask for gluten free) granary toast BBQ Burger – featuring our original barbecue £5.80 Rosemary tossed hand-cut chips £2.70 – homemade with toasted coconut £3.50 sauce recipe and topped with Kino coleslaw Kino muesli Hand-cut sweet potato chips £2.70 flakes, almonds, cashews, apricots, cranberries and Satay Burger – with a rich satay peanut sauce, red £5.80 raisins. -
Bush Tucker Plant Fact Sheets
Traditional Bush Tucker Plant Fact Sheets Acknowledgements: We would like to acknowledge the traditional Noongar owners of this land and custodians of the knowledge used in these Fact Sheets. Illustrations and photos by Melinda Snowball, Deb Taborda, Amy Krupa, Pam Agar and Sian Mawson. ALGAE BUSTER Developed by SERCUL for use with the Bush Tucker Education Program. Used as food Used as medicine Used as resources Local to SW WA Caution: Do not prepare bush tucker food without having been shown by Indigenous or experienced persons. PHOSPHORUS www.sercul.org.au/our-projects/ AWARENESS PROJECT bushtucker/ Some bush tucker if eaten in large quantities or not prepared correctly can cause illness. Australian Bluebell Scientific name: Billardiera heterophylla Aboriginal name: Gumug (Noongar) Plant habit Leaf and stem Flower Fruit About ... Family PITTOSPORACEAE This plant relies on birds to eat the fruit and then Climate Temperate disperse the seeds. The seeds then germinate to produce a new plant. Habitat Open forest and woodland areas Australian bluebells are a common bushland plant Form Small shrub; twiner of the south west of Western Australia. This plant Height: up to 1.5 m has been introduced to the Eastern States, where it is considered a weed; as it forms a thick mat over the Foliage Long, leafy stems which twist around native vegetation. themselves or nearby plants Glossy green, leathery leaves The plant contains toxins which can cause nausea and Length: 50 mm skin irritation, so wear gloves if handling it. (Eurobodalla Shire Council) Flower Birak to Bunuru (Summer) but can flower all year around Intense blue Aboriginal Uses Bell-shaped Occur in clusters of two or more flowers • The fleshy blue berries can be eaten when ripe and Length: up to 10 mm are quite sweet with a soft texture Fruit Follow on from the flower Greenish-blue fruits Length: up to 20 mm Cylindrical in shape Contain many sticky seeds ALGAE BUSTER Developed by SERCUL for use with the Bush Tucker Education Program. -
A HOSPITAL PHARMACOPOEIA of THE- NINETEENTH CENTURY by S
A HOSPITAL PHARMACOPOEIA OF THE- NINETEENTH CENTURY by S. T. ANNING MATTHEWS wrote: 'The compilation of a pharmacopoeia by the physicians and the apothecaries of a hospital proved a great convenience to the many resident and con- sulting physicians treating patients there. Prescriptions in regular use for the treatment of a particular set of symptoms constituted the bulk of the formulae. Time could be saved by merely writing the name of the mixture or pills, etc., required and a supply for stock could be prepared beforehand.... As early as 1730 St. Bartholomew's Hospital, London, had its own printed pharmacopoeia, and, as newly established hospitals adopted their own routines for the dispensing of the medicines ordered for their patients, so the number of hospital pharmacopoeias increased'.' At the General Infirmary at Leeds the earliest pharmacopoeia to be discovered is that of 1863. It is hand-written and the calligraphy throughout is the same but additional entries, presumably later, are in a different hand. There are marginal initialed notes which were written by T.C.A., C.G.W., J.D.H., C.B. and another which is undecipherable. The initials must be those of Thomas Clifford Allbutt,* Claudius Galen Wheelhouse** and John Deakin Heaton***. C.B. has not been identified and was certainly not on the honorary staff of the Infirmary. The marginal notes must have been made between 1864, the year of publication of the first British Pharmacopoeia and of the appointments of Allbutt and Wheelhouse to the staff of the Infirmary, and 1880 when Heaton died. In the Infirmary pharmacopoeia of 1863 there are three Aquae or medicated waters: Aqua Menthol Pip. -
Notes, Outline and Divergence Times of Basidiomycota
Fungal Diversity (2019) 99:105–367 https://doi.org/10.1007/s13225-019-00435-4 (0123456789().,-volV)(0123456789().,- volV) Notes, outline and divergence times of Basidiomycota 1,2,3 1,4 3 5 5 Mao-Qiang He • Rui-Lin Zhao • Kevin D. Hyde • Dominik Begerow • Martin Kemler • 6 7 8,9 10 11 Andrey Yurkov • Eric H. C. McKenzie • Olivier Raspe´ • Makoto Kakishima • Santiago Sa´nchez-Ramı´rez • 12 13 14 15 16 Else C. Vellinga • Roy Halling • Viktor Papp • Ivan V. Zmitrovich • Bart Buyck • 8,9 3 17 18 1 Damien Ertz • Nalin N. Wijayawardene • Bao-Kai Cui • Nathan Schoutteten • Xin-Zhan Liu • 19 1 1,3 1 1 1 Tai-Hui Li • Yi-Jian Yao • Xin-Yu Zhu • An-Qi Liu • Guo-Jie Li • Ming-Zhe Zhang • 1 1 20 21,22 23 Zhi-Lin Ling • Bin Cao • Vladimı´r Antonı´n • Teun Boekhout • Bianca Denise Barbosa da Silva • 18 24 25 26 27 Eske De Crop • Cony Decock • Ba´lint Dima • Arun Kumar Dutta • Jack W. Fell • 28 29 30 31 Jo´ zsef Geml • Masoomeh Ghobad-Nejhad • Admir J. Giachini • Tatiana B. Gibertoni • 32 33,34 17 35 Sergio P. Gorjo´ n • Danny Haelewaters • Shuang-Hui He • Brendan P. Hodkinson • 36 37 38 39 40,41 Egon Horak • Tamotsu Hoshino • Alfredo Justo • Young Woon Lim • Nelson Menolli Jr. • 42 43,44 45 46 47 Armin Mesˇic´ • Jean-Marc Moncalvo • Gregory M. Mueller • La´szlo´ G. Nagy • R. Henrik Nilsson • 48 48 49 2 Machiel Noordeloos • Jorinde Nuytinck • Takamichi Orihara • Cheewangkoon Ratchadawan • 50,51 52 53 Mario Rajchenberg • Alexandre G. -
Plant Exudates and Amber: Their Origin and Uses
Plant Exudates and Amber: Their Origin and Uses Jorge A. Santiago-Blay and Joseph B. Lambert lants produce and export many different some other plant pathology. In other instances, molecules out of their cellular and organ- such as in typical underground roots, exudate Pismal confines. Some of those chemicals production appears to be part of the typical become so abundant that we can see or smell metabolism of healthy plants that helps stabi- them. The most visible materials oozed by lize the soil and foster interactions with other many plants are called “exudates.” organisms around the roots. What are plant exudates? Generally, exudates Different plant tissue types and organs can are carbon-rich materials that many plants pro- produce exudates. We have collected resins and duce and release externally. When exudates are gums from the above ground portions of plants, produced, they are often sticky to human touch. or shoots, as well as from the generally below Such plant chemicals can be the visible expres- ground portion of plants, or roots. Root exuda- sion of attack by bacteria, fungi, herbivores, or tion has been known for decades and is respon- REPRODUCED WITH PERMISSION OF AMERICAN SCIENTIST Resinous exudates on a conifer. ALL PHOTOGRAPHS BY JORGE A. SANTIAGO-BLAY UNLESS OTHERWISE NOTED UNLESS OTHERWISE ALL PHOTOGRAPHS BY JORGE A. SANTIAGO-BLAY Prolific white, resinous exudation is seen on a tumor- Blobs of white resin on a relatively young shoot of a like growth on the trunk of a white pine (Pinus strobus) Japanese black pine (Pinus thunbergii, AA accession at the Arnold Arboretum. -
Supplementary Material
10.1071/BT11174_AC Australian Journal of Botany 60(3), 165–199. CSIRO 2012 Supplementary Material Pollen morphology of the Myrtaceae. Part 1: tribes Eucalypteae, Lophostemoneae, Syncarpieae, Xanthostemoneae and subfamily Psiloxyloideae Andrew H. Thornhill, Geoff S. Hope, Lyn A. Craven and Michael D. Crisp Supplementary Table S1. List of taxa viewed with SEM ANBG - Australian Botanic Gardens, BRI - Queensland Herbarium, CANB, CBG - Australian National Herbarium, CSIRO, Canberra, DNA - Northern Territory Herbarium L - National Herbarium Nederland (Leiden), MI - University of Michigan Herbarium, NSW - National Herbarium of New South Wales, P - Herbier National de Paris. Taxon Voucher details Allosyncarpia ternata S.T.Blake C.R. Dunlop 4626, DNA Angophora costata Britten D. Nicolle 2103, CANB Angophora floribunda (Sm.) Sweet A. Gunnell 18 & W. Bishop, CANB Angophora hispida (Sm.) Blaxell Brooker 12948, CANB Angophora melanoxylon R.T.Baker leg. ign. 841, CANB Arillastrum gummiferum (Brongn. & Gris) Pancher ex Baill. G. McPherson 3580, CANB Corymbia maculata (Hook.) K.D.Hill & L.A.S.Johnson C.R. Dunlop s.n., CANB Corymbia variegata (F. Muell.) K.D.Hill & L.A.S.Johnson M.I.H. Brooker 3360, CANB (Accepted Corymbia citriodora (Hook.) K.D.Hill & L.A.S.Johnson) Eucalyptopsis papuana C.T.White M. Jacobs 9032, CANB Eucalyptus barklyensis L.A.S.Johnson & K.D.Hill K. Hill 3560 & L. Stanberg, DNA Eucalyptus cosmophylla F. Muell. M. Banks 1099, CANB Eucalyptus curtisii Blakely & C.T.White L.H. Bird s.n., NSW Eucalyptus globulus subsp. globulus Labill. T.A. Halliday 609, CANB Eucalyptus gunnii Hook.f. A. Moscal 14907, CANB Eucalyptus haemastoma Sm. R. Coveny 11354 & M.