Controlled Synthesis and Characterization of Ru-Fullerene
Total Page:16
File Type:pdf, Size:1020Kb
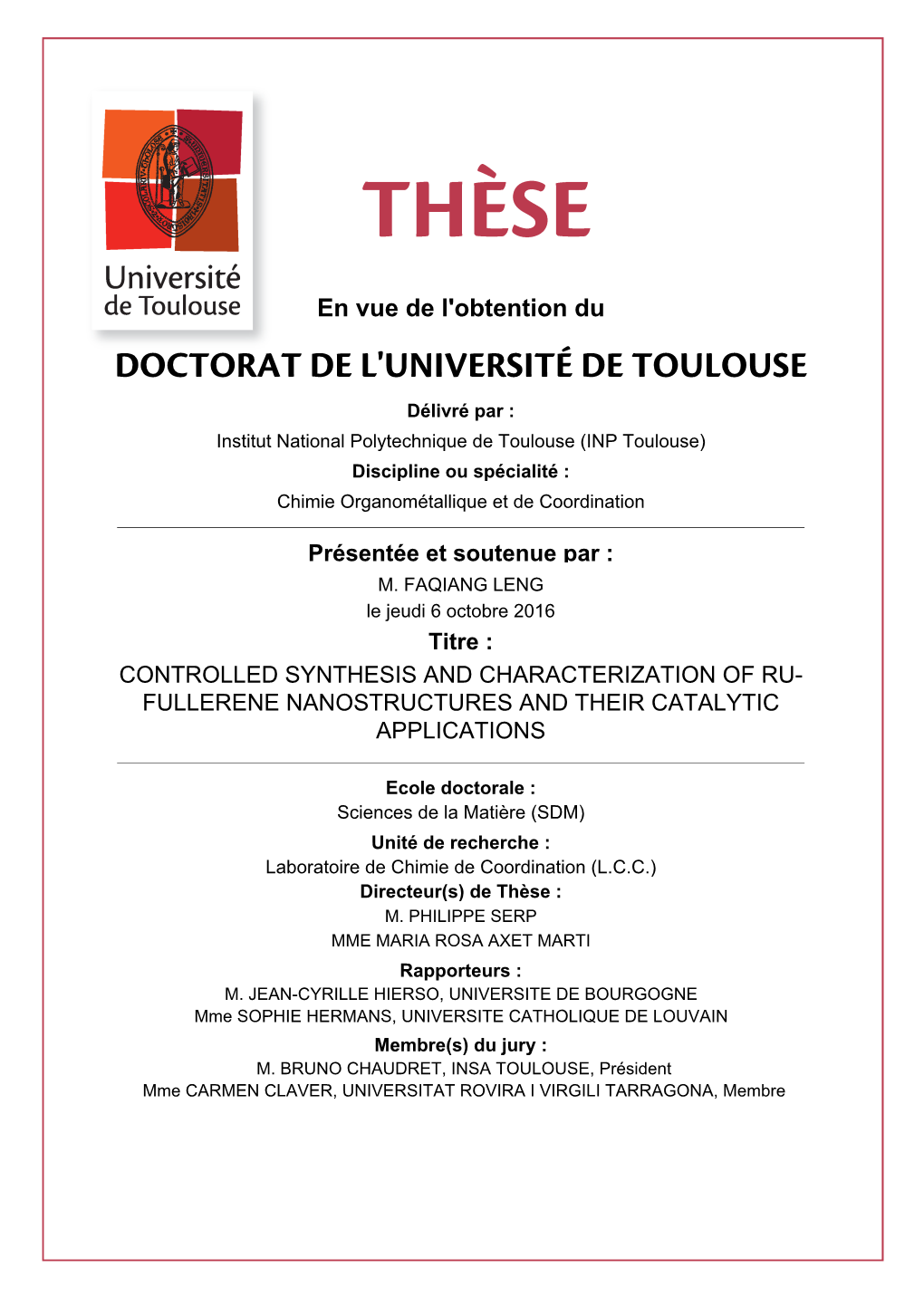
Load more
Recommended publications
-
Deoxygenation Supplemental Without Spectra
Novel Organic Transformations Arising from Gold(I) Chemistry By Mathieu André Morin Thesis submitted to the faculty of Graduate and Postdoctoral Studies In partial fulfillment of the requirements for the Ph.D. degree in chemistry Candidate Supervisor Mathieu André Morin Dr. Louis Barriault Ottawa-Carleton Chemistry Institute Faculty of Science University of Ottawa © Mathieu André Morin, Ottawa, Canada, 2017 Abstract The use of gold in organic chemistry is a relatively recent occurrence. In addition to being previously considered too expensive, it was also believed to be chemically inert. Soon after the early reports indicating its rich reactivity, the number of reports on chemical transformations involving gold sky rocketed. One such report by Toste and coworkers demonstrated the intramolecular addition of silyl enol ether onto Au(I) activated alkynes, resulting in a 5-exo dig cyclization. The first part (Chapter 1) of this thesis discusses the development of a Au(I) catalyzed polycyclization reaction inspired by this transformation. The reaction demonstrated the ability of Au(I) to successfully catalyze the formation of multiple C-C bonds and resulted in the synthesis of benzothiophenes, benzofurans, carbazoles and hydrindene. With the current resurgence of photochemical transformations being reported in literature, various opportunities for the use of Au(I) complexes arose. The substantial relativistic effect observed in gold which make it a good soft Lewis acid also has a significant influence on the redox potential of this metal. Chapter 3 of this thesis discusses the development of a Au(I) photocatalyzed process which benefits from having both a strong oxidation and reduction potential for the reduction of carbon-halide bonds. -
(SAM) Alloy Parts Using X-Ray CT
Research Article ISSN 2639-9466 Nanotechnology & Applications Porosity Determination and Characterization of Binder Jet Printed Structural Amorphous Metal (SAM) Alloy Parts Using X-Ray CT Amamchukwu B. Ilogebe1*, Benedict Uzochukwu2, and Amy M. Elliot3 1North Carolina A&T State University, US. *Correspondence: Amamchukwu B. Ilogebe, North Carolina A&T State University, 2Virginia State University, US. US. 3Oak Ridge National Laboratory, US. Received: 04 November 2019; Accepted: 29 November 2019 Citation: Amamchukwu B. Ilogebe, Benedict Uzochukwu, Amy M. Elliot. Porosity Determination and Characterization of Binder Jet Printed Structural Amorphous Metal (SAM) Alloy Parts Using X-Ray CT. Nano Tech Appl. 2019; 2(1): 1-6. ABSTRACT The advent of Binder jet additive manufacturing continued to a revelation in the manufacture of intricate metal parts. This technology has been utilized in medical, aerospace and automotive industries, not much has been reported in the printing of parts from amorphous metal powders, which have found numerous applications in engineering because of their special properties. In this research, special emphasis was placed on two different manufacturing methods for structural amorphous metal alloy (SAM alloy); Die compaction and Binder jet printing. Samples of SAM alloy was created from these two-manufacturing methods and were subsequently, sintered, analyzed and compared. Previous studies show that as much as up to 50% porosity could be recorded in binder jet printing [1,2]. In this regard, different techniques were used to determine the percentage porosity from both manufacturing methods. The Archimedes method was used to determine the density and percentage porosity of the parts from the two methods. Similarly, percentage porosity was also determined using different tools in computed tomography (CT) analysis. -
Carbon Nanostructures – from Molecules to Functionalised Materials
Digital Comprehensive Summaries of Uppsala Dissertations from the Faculty of Science and Technology 1537 Carbon Nanostructures – from Molecules to Functionalised Materials Fullerene-Ferrocene Oligomers, Graphene Modification and Deposition MICHAEL NORDLUND ACTA UNIVERSITATIS UPSALIENSIS ISSN 1651-6214 ISBN 978-91-513-0019-1 UPPSALA urn:nbn:se:uu:diva-327189 2017 Dissertation presented at Uppsala University to be publicly examined in A1:107a, BMC, Husargatan 3, Uppsala, Friday, 22 September 2017 at 09:15 for the degree of Doctor of Philosophy. The examination will be conducted in English. Faculty examiner: Professor Mogens Brøndsted Nielsen (Copenhagen University, Department of chemistry). Abstract Nordlund, M. 2017. Carbon Nanostructures – from Molecules to Functionalised Materials. Fullerene-Ferrocene Oligomers, Graphene Modification and Deposition. Digital Comprehensive Summaries of Uppsala Dissertations from the Faculty of Science and Technology 1537. 64 pp. Uppsala: Acta Universitatis Upsaliensis. ISBN 978-91-513-0019-1. The work described in this thesis concerns development, synthesis and characterisation of new molecular compounds and materials based on the carbon allotropes fullerene (C60) and graphene. A stepwise strategy to a symmetric ferrocene-linked dumbbell of fulleropyrrolidines was developed. The versatility of this approach was demonstrated in the synthesis of a non- symmetric fulleropyrrolidine-ferrocene-tryptophan triad. A new tethered bis-aldehyde, capable of regiospecific bis-pyrrolidination of a C60-fullerene in predominantly trans fashion, was designed, synthesised and reacted with glycine and C60 to yield the desired N-unfunctionalised bis(pyrrolidine)fullerene. A catenane dimer composed of two bis(pyrrolidine)fullerenes was obtained as a minor co-product. From the synthesis of the N-methyl analogue, the catenane dimer could be separated from the monomeric main product and fully characterised by NMR spectroscopy. -
Measurements of Higher Alkanes Using NO Chemical Ionization in PTR-Tof-MS
Atmos. Chem. Phys., 20, 14123–14138, 2020 https://doi.org/10.5194/acp-20-14123-2020 © Author(s) 2020. This work is distributed under the Creative Commons Attribution 4.0 License. Measurements of higher alkanes using NOC chemical ionization in PTR-ToF-MS: important contributions of higher alkanes to secondary organic aerosols in China Chaomin Wang1,2, Bin Yuan1,2, Caihong Wu1,2, Sihang Wang1,2, Jipeng Qi1,2, Baolin Wang3, Zelong Wang1,2, Weiwei Hu4, Wei Chen4, Chenshuo Ye5, Wenjie Wang5, Yele Sun6, Chen Wang3, Shan Huang1,2, Wei Song4, Xinming Wang4, Suxia Yang1,2, Shenyang Zhang1,2, Wanyun Xu7, Nan Ma1,2, Zhanyi Zhang1,2, Bin Jiang1,2, Hang Su8, Yafang Cheng8, Xuemei Wang1,2, and Min Shao1,2 1Institute for Environmental and Climate Research, Jinan University, 511443 Guangzhou, China 2Guangdong-Hongkong-Macau Joint Laboratory of Collaborative Innovation for Environmental Quality, 511443 Guangzhou, China 3School of Environmental Science and Engineering, Qilu University of Technology (Shandong Academy of Sciences), 250353 Jinan, China 4State Key Laboratory of Organic Geochemistry and Guangdong Key Laboratory of Environmental Protection and Resources Utilization, Guangzhou Institute of Geochemistry, Chinese Academy of Sciences, 510640 Guangzhou, China 5State Joint Key Laboratory of Environmental Simulation and Pollution Control, College of Environmental Sciences and Engineering, Peking University, 100871 Beijing, China 6State Key Laboratory of Atmospheric Boundary Physics and Atmospheric Chemistry, Institute of Atmospheric Physics, Chinese -
The Laser Generation Threshold Characteristics of a Colloidal Solution of Gold and Platinum Nanoparticles with Rodamine 6G
View metadata, citation and similar papers at core.ac.uk brought to you by CORE provided by Tomsk State University Repository 370 17th INTERNATIONAL CONFERENCE ON MICRO/NANOTECHNOLOGIES AND ELECTRON DEVICES EDM 2016 The Laser Generation Threshold Characteristics of a Colloidal Solution of Gold and Platinum Nanoparticles with Rodamine 6G Valeriy A. Donchenko1,2, Mikhail M. Zinoviev1, Alexey A. Zemlyanov2, Vladimir A. Kharenkov1,2, Anna N. Panamaryova3 1National Research Tomsk State University, Tomsk, Russia 2Siberian Physical Technical Institute, Tomsk, Russia 3 National Research Tomsk Polytechnic University, Tomsk, Russia Abstract – In the work the spectral-energy characteristics of or more orders greater than the density of the incident wave stochastic (non-resonator) laser generation of a thin layer of power. organic dye Rhodamine 6G solution with the addition of gold This increases the number of excited molecules within and platinum nanoparticles in the form of a colloidal solution these regions. Due to the Purcell effect [6-8] it is also in ethanol have been experimentally investigated. It has been experimentally established that the generation thresholds possible to increase the speed of active medium molecules are reduced by two orders when adding nanoparticles to the conversions from the excited level into the primary. As active medium. It is shown that the use of nanoparticles having a result, during the agitation action, a large number of a plasmon absorption band in the region of the agitation stimulated emissions of photons appear. This should lead to radiation does not lead to a significant change of the active a decrease in the generation and intensity of emission of the medium generation thresholds. -
A Review of the Use of Nanoparticle Tracking Analysis (NTA)
WHITEPAPER Nanoscale Material Characterization: a Review of the use of Nanoparticle Tracking Analysis (NTA) PARTICLE SIZE Summary PARTICLE This white paper describes the central role of high resolution particle size CONCENTRATION and concentration measurement nanoparticle research. The technique of FLUORESCENCE Nanoparticle Tracking Analysis (NTA) is described and compared to other DETECTION - DELETED characterization methodologies, and comparative papers are cited. A wide range of application studies is then summarized with specific reference to the use and value of NTA. For those seeking a full listing of NTA experience to date by application type, the NanoSight publication “Nanoparticle Tracking Analysis - A review of applications and usage 2010 - 2012, (Carr B and Wright M (2013)) and its successors provides a detailed catalogue. This item is available from your local Malvern Instruments representative or email [email protected]. Malvern Instruments Worldwide Sales and service centres in over 65 countries www.malvern.com/contact ©2017 Malvern Instruments Limited WHITEPAPER Introduction Nanoscale materials, in the form of nanoparticles, are playing an important and growing role across a range of different applications and industries which seek to exploit the unique properties exhibited by these materials, such as their very high surface area to volume ratio and high number. The overall properties and stability of many manufactured products often depends upon the ability to produce particle populations within fine tolerances, without contaminants or aggregates. The concentration of particles within a suspension is another factor that may have an effect upon the desired outcome of the product. It is clear then that there is a real need to characterize a variety of different properties when analyzing nanoparticles, in order to understand the relationship between the formulation and the overall bulk characteristics of the materials (Fedotov, 2011). -
Interactions of Newly Synthesized Platinum Nanoparticles with ICR-191 and Their Potential Application
www.nature.com/scientificreports OPEN Interactions of newly synthesized platinum nanoparticles with ICR- 191 and their potential application Received: 26 November 2018 Agnieszka Borowik1, Rafal Banasiuk2, Natalia Derewonko3, Michal Rychlowski3, Accepted: 4 March 2019 Marta Krychowiak-Masnicka 2, Dariusz Wyrzykowski4, Magdalena Ziabka5, Published: xx xx xxxx Anna Woziwodzka1, Aleksandra Krolicka 2 & Jacek Piosik 1 One of the greatest challenges of modern medicine is to fnd cheaper and easier ways to produce transporters for biologically active substances, which will provide selective and efcient drug delivery to the target cells, while causing low toxicity towards healthy cells. Currently, metal-based nanoparticles are considered a successful and viable solution to this problem. In this work, we propose the use of novel synthesis method of platinum nanoparticles (PtNPs) connected with their precise biophysical characterization and assessment of their potential toxicity. To work as an efcient nanodelivery platform, nanoparticles should interact with the desired active compounds spontaneously and non-covalently. We investigated possible direct interactions of PtNPs with ICR-191, a model acridine mutagen with well-established biophysical properties and mutagenic activity, by Dynamic Light Scattering, fuorescence spectroscopy, and Isothermal Titration Calorimetry. Moreover, to determine the biological activity of ICR-191-PtNPs aggregates, we employed Ames mutagenicity test, eukaryotic cell line analysis and toxicity test against the model -
Controllable ALD Synthesis of Platinum Nanoparticles by Tuning
Journal of Physics D: Applied Physics PAPER Related content - Nucleation and growth process of atomic Controllable ALD synthesis of platinum layer deposition platinum nanoparticles on strontium titanate nanocuboids Chuandao Wang, Linhua Hu, Kenneth nanoparticles by tuning different synthesis Poeppelmeier et al. parameters - Atomic layer deposition of Pd and Pt nanoparticles for catalysis: on the mechanisms of nanoparticle formation To cite this article: Chuandao Wang et al 2017 J. Phys. D: Appl. Phys. 50 415301 Adriaan J M Mackus, Matthieu J Weber, Nick F W Thissen et al. - Sub-nanometer dimensions control of core/shell nanoparticles prepared by atomic layer deposition View the article online for updates and enhancements. M J Weber, M A Verheijen, A A Bol et al. This content was downloaded from IP address 129.105.122.65 on 04/12/2017 at 17:10 IOP Journal of Physics D: Applied Physics Journal of Physics D: Applied Physics J. Phys. D: Appl. Phys. J. Phys. D: Appl. Phys. 50 (2017) 415301 (9pp) https://doi.org/10.1088/1361-6463/aa8709 50 Controllable ALD synthesis of platinum 2017 nanoparticles by tuning different synthesis © 2017 IOP Publishing Ltd parameters JPAPBE Chuandao Wang1,2,4, Linhua Hu2, Yuyuan Lin1,2, Kenneth Poeppelmeier2, Peter Stair2,3 and Laurence Marks1 415301 1 Department of Materials Science and Engineering, Northwestern University, 2220 North Campus Drive, Evanston, IL 602083108, United States of America C Wang et al 2 Department of Chemistry, Northwestern University, 2145 Sheridan Road, Evanston, IL 602083113, United States of America 3 Chemical Sciences and Engineering Division, Argonne National Laboratory, 9700 South Cass Avenue, Argonne, IL 60439, United States of America Printed in the UK Email: [email protected] Received 17 July 2017, revised 11 August 2017 JPD Accepted for publication 18 August 2017 Published 15 September 2017 10.1088/1361-6463/aa8709 Abstract Pt nanoparticles were successfully deposited using three different atomic layer deposition (ALD) methods, e.g. -
Synthesis of Novel Fullerene Architectures with Mixed Octahedral Addition Pattern Synthese Neuartiger Fulleren Architekturen
Synthesis of Novel Fullerene Architectures with Mixed Octahedral Addition Pattern Synthese neuartiger Fulleren Architekturen mit gemischtem oktaedrischen Additionsmuster Der Naturwissenschaftlichen Fakultät der Friedrich-Alexander-Universität Erlangen-Nürnberg zur Erlangung des Doktorgrades Dr. rer. nat. vorgelegt von Ekaterini Vlassiadi aus Nürnberg Als Dissertation genehmigt von der Naturwissenschaftlichen Fakultät der Friedrich-Alexander-Universität Erlangen-Nürnberg Tag der mündlichen Prüfung: 24.07.2017 Vorsitzender der Promotionskommission: Prof. Dr. Georg Kreimer Gutachter: Prof. Dr. Andreas Hirsch Prof. Dr. Jürgen Schatz Mein besonderer Dank gilt meinem Doktorvater Prof. Dr. Andreas Hirsch für die Bereitstellung des interessanten und herausfordernden Themas, seine Förderung, die fachliche Unterstützung und das Interesse am Fortgang meiner Forschungsarbeit. Die vorliegende Arbeit entstand in der Zeit von November 2012 bis Dezember 2016 am Lehrstuhl für Organische Chemie II des Departments für Chemie und Pharmazie der Friedrich-Alexander-Universität Erlangen-Nürnberg. Table of Contents 1. Introduction 1 1.1. Discovery of Fullerenes 1 1.2. Structure of C60 2 1.3. Physical and Spectroscopic Properties of C60 3 1.3.1. Solubility 3 1.3.2. Mass Spectrometry 4 1.3.3. NMR Spectroscopy 4 1.3.4. UV/Vis Spectroscopy 6 1.4. Electronic Properties and Chemical Reactivity of [60] Fullerene 7 1.5. Chemical reactivity of Fullerenes 10 1.5.1. Endohedral Functionalization of C60 10 1.5.2. Fulleride Salts 11 1.5.3. Heterofullerenes 12 1.5.4. Open-Shell Fullerene Fragments 12 1.5.5. Exohedral Functionalization of C60 13 1.5.5.1 Addition of Nucleophiles 13 1.5.5.2. Cycloaddition reactions 15 1.6. Multiple Exohedral Functionalization 18 1.6.1 Introduction to the Nomenclature of Multiple-Adducts 18 1.6.2. -
Process, Structure, Property and Applications of Metallic Glasses
AIMS Materials Science, 3(3): 1022-1053. DOI: 10.3934/matersci.2016.3.1022 Received: 15 March 2016 Accepted: 07 July 2016 Published: 26 July 2016 http://www.aimspress.com/journal/Materials Short review Process, structure, property and applications of metallic glasses Bindusri Nair and B. Geetha Priyadarshini * Nanotech Research Innovation and Incubation Centre, PSG Institute of Advanced Studies, Coimbatore, Tamil Nadu, India-641004 * Correspondence: Email: [email protected]. Abstract: Metallic glasses (MGs) are gaining immense technological significance due to their unique structure-property relationship with renewed interest in diverse field of applications including biomedical implants, commercial products, machinery parts, and micro-electro-mechanical systems (MEMS). Various processing routes have been adopted to fabricate MGs with short-range ordering which is believed to be the genesis of unique structure. Understanding the structure of these unique materials is a long-standing unsolved mystery. Unlike crystalline counterpart, the outstanding properties of metallic glasses owing to the absence of grain boundaries is reported to exhibit high hardness, excellent strength, high elastic strain, and anti-corrosion properties. The combination of these remarkable properties would significantly contribute to improvement of performance and reliability of these materials when incorporated as bio-implants. The nucleation and growth of metallic glasses is driven by thermodynamics and kinetics in non-equilibrium conditions. This comprehensive review article discusses the various attributes of metallic glasses with an aim to understand the fundamentals of relationship process-structure-property existing in such unique class of material. Keywords: metallic glasses; glass transition; amorphous; mechanical properties 1. Introduction Metallic Glasses (MG) are a class of materials which has caught the eye of many researchers since Klement et al’s [1] first work on Au-Si alloys in early 1960’s. -
Mechanical Behavior of Amorphous Alloys
Acta Materialia 55 (2007) 4067–4109 www.elsevier.com/locate/actamat Overview No. 144 Mechanical behavior of amorphous alloys Christopher A. Schuh a,*, Todd C. Hufnagel b, Upadrasta Ramamurty c a Department of Materials Science and Engineering, Massachusetts Institute of Technology, 77 Massachusetts Avenue, M.I.T., Cambridge, MA 02139, USA b Department of Materials Science and Engineering, Johns Hopkins University, Baltimore, MD 21218, USA c Department of Materials Engineering, Indian Institute of Science, Bangalore-560 012, India Received 14 August 2006; received in revised form 29 January 2007; accepted 31 January 2007 Available online 19 April 2007 Abstract The mechanical properties of amorphous alloys have proven both scientifically unique and of potential practical interest, although the underlying deformation physics of these materials remain less firmly established as compared with crystalline alloys. In this article, we review recent advances in understanding the mechanical behavior of metallic glasses, with particular emphasis on the deformation and fracture mechanisms. Atomistic as well as continuum modeling and experimental work on elasticity, plastic flow and localization, frac- ture and fatigue are all discussed, and theoretical developments are connected, where possible, with macroscopic experimental responses. The role of glass structure on mechanical properties, and conversely, the effect of deformation upon glass structure, are also described. The mechanical properties of metallic glass-derivative materials – including in situ and ex situ composites, foams and nanocrystal- reinforced glasses – are reviewed as well. Finally, we identify a number of important unresolved issues for the field. Ó 2007 Acta Materialia Inc. Published by Elsevier Ltd. All rights reserved. Keywords: Metallic glass; Amorphous metal; Mechanical properties 1. -
The R3-Carbon Allotrope
The R3-carbon allotrope: a pathway towards glassy carbon under high SUBJECT AREAS: MECHANICAL pressure PROPERTIES ELECTRONIC MATERIALS Xue Jiang1,2, Cecilia A˚ rhammar3, Peng Liu1, Jijun Zhao2 & Rajeev Ahuja1,4 STRUCTURE OF SOLIDS AND LIQUIDS 1Department of Materials and Engineering, Royal Institute of Technology, 10044 Stockholm, Sweden, 2Key Laboratory of Materials ELECTRONIC STRUCTURE Modification by Laser, Ion and Electron Beams Dalian University of Technology, Ministry of Education, Dalian 116024, China, 3Sandvik Coromant, Lerkrogsv. 13, S-126 80 Stockholm, Sweden, 4Department of Physics and Astronomy, Box 516, Uppsala University, 75120, Uppsala, Sweden. Received 8 November 2012 Pressure-induced bond type switching and phase transformation in glassy carbon (GC) has been simulated Accepted by means of Density Functional Theory (DFT) calculations and the Stochastic Quenching method (SQ) in a 25 April 2013 wide range of pressures (0–79 GPa). Under pressure, the GC experiences a hardening transition from sp- and sp2-type to sp3-type bonding, in agreement with previous experimental results. Moreover, a new Published crystalline carbon allotrope possessing R3 symmetry (R3-carbon) is predicted using the stochastic SQ 23 May 2013 method. The results indicate that R3-carbon can be regarded as an allotrope similar to that of amorphous GC. A very small difference in the heat of formation and the coherence of the radial and angular distribution functions of GC and the R3-carbon structure imply that small perturbations to this crystalline carbon Correspondence and allotrope may provide another possible amorphization pathway of carbon besides that of quenching the liquid melt or gas by ultra-fast cooling. requests for materials should be addressed to J.Z.