127-137 Distribution of Rees and Yttrium Among Major Geochemical
Total Page:16
File Type:pdf, Size:1020Kb
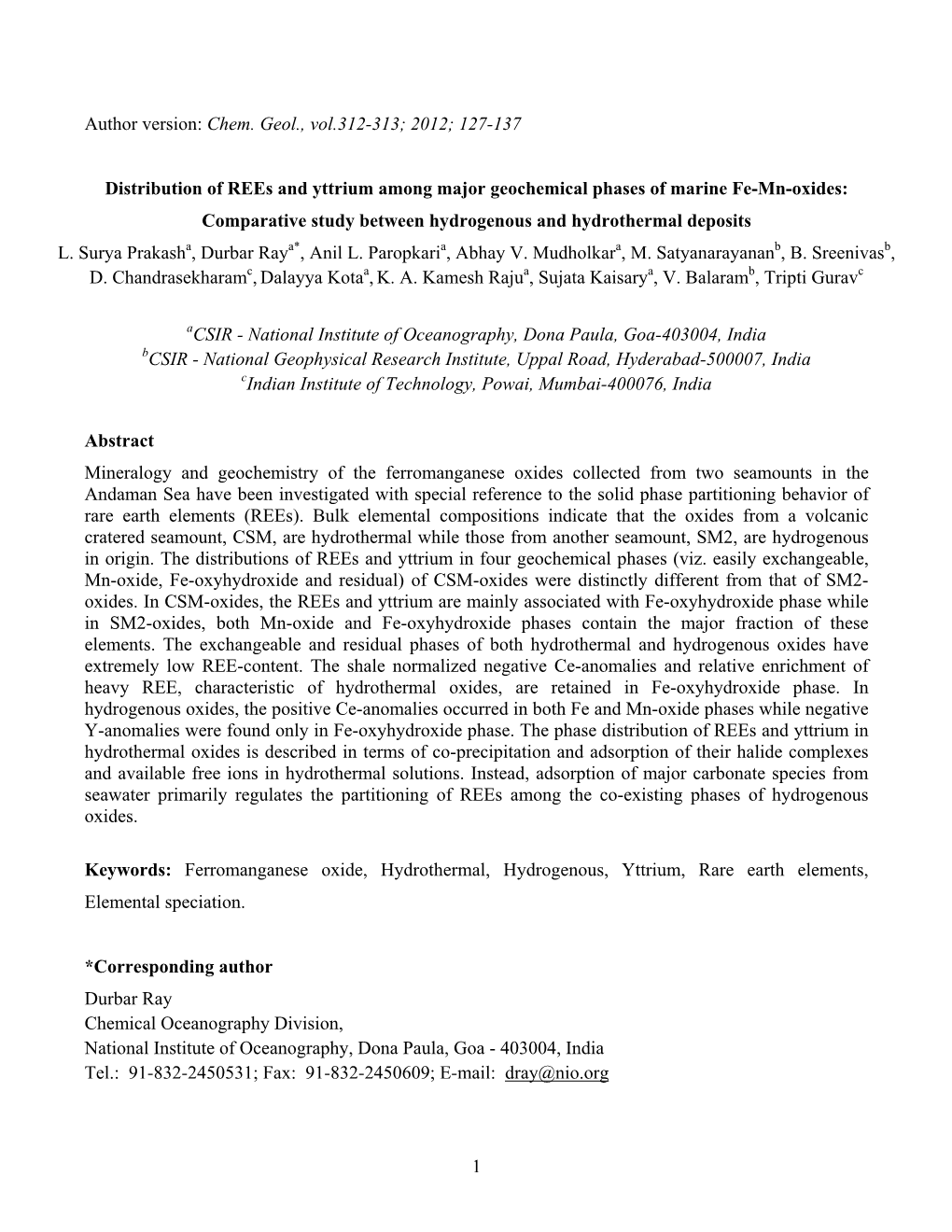
Load more
Recommended publications
-
Université Du Québec À Chicoutimi
UNIVERSITÉ DU QUÉBEC À CHICOUTIMI MÉMOIRE PRÉSENTE À L'UNIVERSITÉ DU QUÉBEC À CHICOUTIMI COMME EXIGENCE PARTIELLE DE LA MAÎTRISE EN SCIENCES DE LA TERRE PAR ALEXANDRE AUBIN BSc. Ing THE SCHAKALSBERG SEAMOUNT: PHYSICAL VOLCANOLOGY, STRUCTURE, ALTERATION AND MINERALIZATION FEVRIER 2004 UIUQAC bibliothèque Paul-Emile-Bouletj Mise en garde/Advice Afin de rendre accessible au plus Motivated by a desire to make the grand nombre le résultat des results of its graduate students' travaux de recherche menés par ses research accessible to all, and in étudiants gradués et dans l'esprit des accordance with the rules règles qui régissent le dépôt et la governing the acceptation and diffusion des mémoires et thèses diffusion of dissertations and produits dans cette Institution, theses in this Institution, the l'Université du Québec à Université du Québec à Chicoutimi (UQAC) est fière de Chicoutimi (UQAC) is proud to rendre accessible une version make a complete version of this complète et gratuite de cette œuvre. work available at no cost to the reader. L'auteur conserve néanmoins la The author retains ownership of the propriété du droit d'auteur qui copyright of this dissertation or protège ce mémoire ou cette thèse. thesis. Neither the dissertation or Ni le mémoire ou la thèse ni des thesis, nor substantial extracts from extraits substantiels de ceux-ci ne it, may be printed or otherwise peuvent être imprimés ou autrement reproduced without the author's reproduits sans son autorisation. permission. 11 RÉSUMÉ Les montagnes du Schakalsberg du Sperrgebiet en Namibie sont considérées comme partie intégrante de la Ceinture Néo protérozoïque de Gariep (745-550 Ma), qui est connue pour le gisement de métaux de base de Rosh Pinah (30 millions de tonnes, Zn-Pb- Cu-Ag). -
Cu–Mn–Fe Alloys and Mn-Rich Amphiboles in Ancient Copper Slags
Journal of African Earth Sciences 101 (2015) 70–83 Contents lists available at ScienceDirect Journal of African Earth Sciences journal homepage: www.elsevier.com/locate/jafrearsci Cu–Mn–Fe alloys and Mn-rich amphiboles in ancient copper slags from the Jabal Samran area, Saudi Arabia: With synopsis on chemistry of Fe–Mn(III) oxyhydroxides in alteration zones ⇑ Adel A. Surour Department of Mineral Resources and Rocks, Faculty of Earth Sciences, King Abdulaziz University, P.O. Box 80206, Jeddah 21589, Saudi Arabia Department of Geology, Faculty of Science, Cairo University, Egypt article info abstract Article history: In the Jabal Samran area (western Saudi Arabia), secondary copper mineralization in a NE-trending shear Received 15 July 2014 zone in which the arc metavolcanic host rocks (dacite–rhyodacite) show conjugate fractures and exten- Received in revised form 27 August 2014 sive hydrothermal alteration and bleaching. The zones contain frequent Fe–Mn(III) oxyhydroxides Accepted 2 September 2014 (FeOH–MnOH) that resulted from oxidation of pyrite and Mn-bearing silicates. In the bleached part, Available online 16 September 2014 the groundmass is represented by Fe-bearing interstratified illite–smectite with up to 4.02 wt% FeOt. FeOH–MnOH are pre-weathering phases formed by hydrothermal alteration in a submarine environment Keywords: prior to uplifting. Five varieties of FeOH are distinguished, four of them are exclusively hydrothermal Fe–Mn(III) oxyhydroxide with 20 wt% H O whereas the fifth contains 31–33 wt% H O and might represent reworking of earlier Synthetic Mn-rich amphiboles 2 2 Cu prills hydrothermal FeOH phases by weathering. FeOH fills thin fractures in the form of veinlets and crenulated Slags laminae or as a pseudomorph for pyrite, goethite and finally ferrihydrite, and this oxyhydroxide is char- acterized by positive correlation of Fe2O3 with SiO2 and Al2O3. -
Diffuse, Low-Temperature Hydrothermal Deposits on the Juan De Fuca Ridge and Plate
DIFFUSE, LOW-TEMPERATURE HYDROTHERMAL DEPOSITS ON THE JUAN DE FUCA RIDGE AND PLATE Catherine Erma Channing B.Sc., Carleton University, 2001 A Thesis Submitted in Partial Fulfillment of the Requirements for the Degree of MASTER OF SCIENCE In the Department of Earth and Ocean Sciences O Catherine E. Channing, 2004 University of Victoria All rights reserved. This thesis may not be reproduced in whole or in part, by photocopy or other means, without permission of the author. Supervisor: Dr. Kathryn M. Gillis ABSTRACT Hydrothermal circulation in ocean crust results in significant geochemical exchanges between hydrosphere and lithosphere. This process begins at the mid-ocean ridge and continues as basaltic crust ages and is subducted, significantly altering the chemical composition of both fluid and rock. In the on-axis environment, heated crustal fluids with a composition altered from that of seawater vent as either high temperature (> 100 C), focused flow or low temperature (< 100 oC) diffuse flow. Reaction between warm fluids and basalt results in the alteration of the rock, manifested as the breakdown of glass and primary minerals and the deposition of secondary minerals. In the off-axis environment (crust > 1 Ma), crustal fluids discharge locally at seamounts, where extensive manganese oxides can precipitate. Both types of mineral deposits record the time-integrated history of diffuse fluid-rock interaction, and in addition, Mn-oxide deposits are useful for estimating the longevity of hydrothermal activity. The effects of low-temperature diffuse fluids on the basaltic crust was examined at both young (Axial Volcano) and mature (Main Endeavour field) on-axis hydrothermal sites. -
Download (1614Kb)
Received: 15 October 2019 | Revised: 31 March 2020 | Accepted: 27 April 2020 DOI: 10.1111/ter.12468 RESEARCH ARTICLE Origin of isolated seamounts in the Canary Basin (East Atlantic): The role of plume material in the origin of seamounts not associated with hotspot tracks Xiaojun Long1 | Jörg Geldmacher1 | Kaj Hoernle1,2 | Folkmar Hauff1 | Jo-Anne Wartho1 | C.-Dieter Garbe-Schönberg2 1GEOMAR Helmholtz Centre for Ocean Research Kiel, Kiel, Germany Abstract 2Institute of Geosciences, Kiel University, In contrast to seamount chains, small solitary seamounts/seamount groups have Kiel, Germany rarely been sampled despite their large number and therefore their origins remain 40 39 Correspondence enigmatic. Here we present new Ar/ Ar, trace element and Nd-Hf-Pb isotope data Xiaojun Long, GEOMAR Helmholtz Centre from the solitary Demenitskoy Seamount, the isolated Tolkien seamount group and for Ocean Research Kiel, Wischhofstrasse 1-3, 24148 Kiel, Germany. the Krylov Seamount and Ridge in the Canary Basin, Central Atlantic Ocean. Their Email: [email protected] chemical compositions range from intraplate ocean-island-basalt (Demenitskoy) to Funding information mid-ocean-ridge-basalt (Tolkien and Krylov) types. Lavas from all three seamount China Scholarship Council, Grant/Award groups, however, show geochemical evidence for involvement of enriched Canary/ Number: [2015]0672 Cape Verde plume material. Seismic tomography shows that large areas around these mantle plumes consist of dispersed low-velocity material, which could represent dif- fusely-upwelling plume mantle. Melts from such upwelling mantle could form isolated seamounts. Diffuse upwelling of plume material is likely to be extremely widespread but has been poorly studied to date. Significance Statement: A fundamental question concerns the origin of the hundreds of thousands of solitary seamounts and small isolated clusters of such seamounts on the seafloor of the world's ocean basins. -
Rare Earth Element Geochemistry of the Sediments, Ferromanganese Nodules and Crusts from the Indian Ocean
RARE EARTH ELEMENT GEOCHEMISTRY OF THE SEDIMENTS, FERROMANGANESE NODULES AND CRUSTS FROM THE INDIAN OCEAN SUBMITTED TO THE GOA UNIVERSITY FOR THE DEGREE OF DOCTOR OF PHILOSOPHY BY BEJUGAM NAGENDER NATH NATIONAL INSTITUTE OF OCEANOGR RESEARCH GUIDE I „ R.R. NAIR \ 111 DEPUTY DIRECTOR AND HEA 0 A ..30"" GEOLOGICAL OCEANOGRAPHY DIVISION- 5-5 1 .46 N f / RAd NATIONAL INSTITUTE OF OCEANOGRAPHY DONA PAULA, GOA - 403 004, INDIA. OCTOBER, 1993 "THESE ELEMENTS (REE) PERPLEX US IN OUR RESEARCHES, BAFFLE US IN OUR SPECULATIONS, AND HAUNT US IN OUR VERY DREAMS. THEY STRETCH LIKE AN UNKNOWN SEA BEFORE US - MOCKING, MYSTIFYING AND MURMURING STRANGE REVELATIONS AND POSSIBILITIES" SIR WILLIAM CROOKES, 1887 (as quoted in Elderfield, 1988) CERTIFICATE Mr. B. Nagender Nath has been working under my guidance since 1991. The Ph.D. thesis entitled "Rare earth element geochemistry of the sediments, ferromanganese nodules and crusts from the Indian Ocean", submitted by him contains the results of his original investigation of the subject. This is to certify that the thesis has not been the basis for the award of any other research degree or diploma of other University. ____.---,_ --- 0 ".„..--,.... ., o. ( :At \ / ( R. R. NAIR ) \\N \s \ ." ..„_. __- -*/ Research Guide ,,, G_ 0 puty Director and Head, Geb j eanography Division National Institute of Oceanography Dona Paula, GOA - 403 004. CONTENTS CONTENTS Page No. ACKNOWLEDGEMENTS LIST OF FIGURES iv-xi LIST OF TABLES xii-xiii CHAPTER 1 1-23 INTRODUCTION 1.1. Occurrence and abundance 2 1.2. Historical aspects 3 1.3. General chemistry of REE 5 1.31. -
Natural Speciation of Mn, Ni and Zn at the Micrometer Scale in a Clayey Paddy Soil Using X-Ray Fluorescence, Absorption, and Diffraction
Natural speciation of Mn, Ni and Zn at the micrometer scale in a clayey paddy soil using X-ray fluorescence, absorption, and diffraction. Alain Manceau, Caterina Tommaseo, Sophie Rihs, Nicolas Geoffroy, Daniel Chateigner, Michel Schlegel, Delphine Tisserand, Matthew A. Marcus, Nobumichi Tamura, Zueng-Sang Chen To cite this version: Alain Manceau, Caterina Tommaseo, Sophie Rihs, Nicolas Geoffroy, Daniel Chateigner, et al.. Natural speciation of Mn, Ni and Zn at the micrometer scale in a clayey paddy soil using X-ray fluorescence, absorption, and diffraction.. Geochimica et Cosmochimica Acta, Elsevier, 2005, 69/16, pp.4007-4034. 10.1016/j.gca.2005.03.018. hal-00107092 HAL Id: hal-00107092 https://hal.archives-ouvertes.fr/hal-00107092 Submitted on 17 Oct 2006 HAL is a multi-disciplinary open access L’archive ouverte pluridisciplinaire HAL, est archive for the deposit and dissemination of sci- destinée au dépôt et à la diffusion de documents entific research documents, whether they are pub- scientifiques de niveau recherche, publiés ou non, lished or not. The documents may come from émanant des établissements d’enseignement et de teaching and research institutions in France or recherche français ou étrangers, des laboratoires abroad, or from public or private research centers. publics ou privés. 1 Natural speciation of Mn, Ni and Zn at the micrometer scale in a clayey paddy soil using X-ray fluorescence, absorption, and diffraction Alain Manceau1,*, Caterina Tommaseo1, Sophie Rihs2, Nicolas Geoffroy1, Daniel Chateigner3, Michel Schlegel4, Delphine Tisserand1, Matthew A. Marcus5, Nobumichi Tamura5, Zueng-Sang Chen6 1 Environmental Geochemistry Group, Maison des Géosciences, Univ. J. Fourier, BP 53, 38041 Grenoble Cedex 9, France. -
Ifm-Geomar Report
FS POSEIDON Fahrtbericht / Cruise Report P379/2 Mid-Atlantic-Researcher Ridge Volcanism (MARRVi) Mindelo - Fort-de-France 15.02.-08.03.2009 IFM-GEOMAR REPORT Berichte aus dem Leibniz-Institut für Meereswissenschaften an der Christian-Albrechts-Universität zu Kiel Nr. 29 June 2009 FS POSEIDON Fahrtbericht / Cruise Report P379/2 Mid-Atlantic-Researcher Ridge Volcanism (MARRVi) Mindelo - Fort-de-France 15.02.-08.03.2009 Berichte aus dem Leibniz-Institut für Meereswissenschaften an der Christian-Albrechts-Universität zu Kiel Nr. 29, June 2009 ISSN Nr.: 1614-6298 Das Leibniz-Institut für Meereswissenschaften The Leibniz-Institute of Marine Sciences is a ist ein Institut der Wissenschaftsgemeinschaft member of the Leibniz Association Gottfried Wilhelm Leibniz (WGL) (Wissenschaftsgemeinschaft Gottfried Wilhelm Leibniz). Herausgeber / Editor: Svend Duggen IFM-GEOMAR Report ISSN Nr.: 1614-6298 Leibniz-Institut für Meereswissenschaften / Leibniz Institute of Marine Sciences IFM-GEOMAR Dienstgebäude Westufer / West Shore Building Düsternbrooker Weg 20 D-24105 Kiel Germany Leibniz-Institut für Meereswissenschaften / Leibniz Institute of Marine Sciences IFM-GEOMAR Dienstgebäude Ostufer / East Shore Building Wischhofstr. 1-3 D-24148 Kiel Germany Tel.: ++49 431 600-0 Fax: ++49 431 600-2805 www.ifm-geomar.de Cruise Report POS379/2 (MARRVi) – MAR-Researcher Ridge 15. Feb. – 8. Mrz. 2009 Table of Contents Chapter 1: Scientific Party and Crew 2 Chapter 2: Introduction and Scientific Background 3 Chapter 3: Methods 8 3.1. The ELAC multi-beam system 3.2. The chain sack dredge 3.3. Magnetotellurics Chapter 4: Regional Geology and Preliminary Results 12 4.1. Isolated Seamounts 4.2. Mid-Atlantic Ridge 4.3. Researcher Ridge Chapter 5: Cruise Narrative 17 Chapter 6: Station Summary 23 Chapter 7: Sample Description 25 7.1. -
Investigating the Potential Recovery of REY from Metalliferous Sediments in a Seafloor Analogue; the Troodos Ophiolite, Cyprus
UNIVERSITY OF SOUTHAMPTON Faculty of Natural and Environmental Sciences Ocean and Earth Science Investigating the potential recovery of REY from metalliferous sediments in a seafloor analogue; The Troodos ophiolite, Cyprus by Pierre Josso Thesis for the degree of Doctor of Philosophy Submitted 16th of January 2017 UNIVERSITY OF SOUTHAMPTON ABSTRACT FACULTY OF NATURAL AND ENVIRONMENTAL SCIENCES Ocean and Earth Science Geochemistry Thesis for the degree of Doctor of Philosophy INVESTIGATING THE POTENTIAL RECOVERY OF REY FROM METALLIFEROUS SEDIMENTS IN A SEAFLOOR ANALOGUE; THE TROODOS OPHIOLITE, CYPRUS Pierre Josso The perceived supply risk for essential materials used in the development of green energy and other state-of-the art technologies creates the need for investigation of new sources for these raw materials. Many of these raw materials are characterized as “critical” given supply risks posed by geographic location, the economic and political stability of producing countries, potential substitution and opportunities for recycling [European Commission, 2014]. At present, 20 raw materials are listed by the EU as critical and this inventory is likely to grow in the coming years as the world population increases, driven by the development of India, China, Africa, Brazil and others. Among these critical elements, the rare earth elements and yttrium (REY) form a group of 15 metals essential for the development of wind turbines, cell phones and batteries among other applications and their production has been under Chinese domination for the last three decades. More than 95 % of the consumed REY worldwide originated in China during the last thirty years, a monopole that reflects economical constrains rather than the unequal distribution of REY resources across the world. -
Ore Geology Reviews 87 (2017) 41–61
Ore Geology Reviews 87 (2017) 41–61 Contents lists available at ScienceDirect Ore Geology Reviews journal homepage: www.elsevier.com/locate/oregeorev Strategic and rare elements in Cretaceous-Cenozoic cobalt-rich ferromanganese crusts from seamounts in the Canary Island Seamount Province (northeastern tropical Atlantic) E. Marino a,b,c,⁎,F.J.Gonzáleza, L. Somoza a,R.Lunarb,c,L.Ortegab,J.T.Vázquezd,J.Reyesa,E.Bellidoa a Geological Survey of Spain, (IGME), C/Ríos Rosas, 23, 28003 Madrid, Spain b Crystallography and Mineralogy Department, Complutense University of Madrid, C/Jose Antonio Novais, 2, 28040 Madrid, Spain c Instituto de Geociencias IGEO (CSIC, UCM), Madrid, Spain d Instituto Español de Oceanografía, C.O. de Málaga, Puerto Pesquero s/n, 29649 Fuengirola, Spain article info abstract Article history: Thick ferromanganese (Fe-Mn) crusts from four Cretaceous seamounts (The Paps, Tropic, Echo and Drago) at the Received 1 April 2016 southern Canary Island Seamount Province (CISP) in the northeastern tropical Atlantic were recovered along the Received in revised form 20 September 2016 flanks and summits from 1700 to 3000 m water depths. CISP is composed of N100 seamounts and submarine Accepted 7 October 2016 hills, is likely the oldest hotspot track in the Atlantic Ocean, and is the most long-lived of known hotspots globally. Available online 13 October 2016 The Fe-Mn crusts grow on basalt-sedimentary rock substrates below the northeastern tropical Atlantic core of the oxygen minimum zone (OMZ) with a maximum thickness of 250 mm at a water depth of 2400 m. The mineral- Keywords: Ferromanganese crusts ogical and chemical composition of these Fe-Mn crusts indicate a hydrogenetic origin. -
DEPARTMENT of the INTERIOR U.S. GEOLOGICAL SURVEY Geological, Geochemical, Geophysical, and Oceanographic Data and Interpretatio
DEPARTMENT OF THE INTERIOR U.S. GEOLOGICAL SURVEY Geological, Geochemical, Geophysical, and Oceanographic Data and Interpretations of Seamounts and Co-rich Ferromanganese Crusts from the Marshall Islands, KORDI- USGS R.V. Farnella Cruise F10-89-CP by James R. Heinl, Jung-Keuk Kang2, Marjorie S. Schulz*, Byong-Kwon Park2, Herbert Kirschenbaum3, Suk-Hoon Yoon2, Renee L. Olsonl, Virginia K. Smith*, Dong-Won Park2, George O. Riddle*, Paula J. Quinternol, Yoon-Oh Lee5, Alice S. Davis1 , Seong Ryul Kim2, Malcolm S. Pringle*, Dong-Lim Choi2, LedaBeth Pickthornl, Seymour O. Schlanger6, Frederick K. Duennebier?, Douglas D. Bergersen?, and Jonathan M. Lincoln6 Open File Report 90-407 This report is preliminary and has not been reviewed for conformity with the U.S. Geological Survey editorial standards or with the North American Stratigraphic Code. Any use of trade, product, or firm names is for descriptive purposes only and does not imply endorsement by the U.S. Government. 1U.S. Geological Survey, Menlo Park, CA 2Korea Ocean Research and Development Institute, Seoul 3U.S. Geological Survey, Reston, VA 4U.S. Geological Survey, Denver, CO 5Korea Research Institute of Energy and Resources, Seoul ^Northwestern University, Evanston, IL ^University of Hawaii, Honolulu, HI INTRODUCTION From 6 September to 3 October 1989, the Korea Ocean Research and Development Institute (KORDI) and the U.S. Geological Survey (USGS) participated in a cooperative cruise (F10-89-CP) to the Marshall Islands (Figs. 1, 2). Eight scientists from Korea, twelve from the United States, and one observer from the Republic of the Marshall Islands comprised the scientific staff (Table 1). The two main purposes of this cruise were: 1. -
Polymetallic Massive Sulphide Deposits at the Modern Seafloor
Polymetallic Massive Sulphides and Cobalt-Rich Ferromanganese Crusts: Status and Prospects ISA Technical Study: No.2 International Seabed Authority Polymetallic Massive Sulphides and Cobalt-Rich Ferromanganese Crusts: Status and Prospects This report contains the full text of four presentations originally given at an international workshop on deep ocean mineral resources beyond the limits of national jurisdiction held in Kingston, Jamaica in June 2000. International Seabed Authority 3 The designations employed and the presentation of material in this publication do not imply the expression of any opinion whatsoever on the part of the Secretariat of the International Seabed Authority concerning the legal status of any country or territory or of its authorities, or concerning the delimination of its frontiers or maritime boundaries. All rights reserved. No part of this publication may be reproduced, stored in a retrieval system, or transmitted in any form or by any means, electronic, mechanical, photocopying or otherwise, without the prior permission of the copyright owner. Applications for such permission with a statement of purpose and extent of the reproduction should be addressed to the Secretary-General, International Seabed Authority, 14-20 Port Royal Street, Kingston, Jamaica. National Library of Jamaica Cataloguing-in-Publication Data Polymetallic massive sulphides and cobalt-rich ferromanganese crusts : status and prospects. p. : ill., maps; cm. – (ISA technical study ; no.2) Includes bibliography. ISBN: 976-610-467-0 1. Oceanography -
Hydrogenetic, Diagenetic and Hydrothermal Processes Forming Ferromanganese Crusts in the Canary Island Seamounts and Their Influ
minerals Article Hydrogenetic, Diagenetic and Hydrothermal Processes Forming Ferromanganese Crusts in the Canary Island Seamounts and Their Influence in the Metal Recovery Rate with Hydrometallurgical Methods Egidio Marino 1,2,3,* , Francisco Javier González 1 , Thomas Kuhn 4 , Pedro Madureira 5, Anna V. Wegorzewski 4, Jose Mirao 5, Teresa Medialdea 1 , Martin Oeser 6, Catarina Miguel 5, Jesús Reyes 7, Luis Somoza 1 and Rosario Lunar 2,3 1 Marine Geology Division, Geological Survey of Spain (IGME), Ríos Rosas, 23, 28003 Madrid, Spain 2 Mineralogy and Petrology Department, Geology Faculty, Complutense University of Madrid (UCM), Jose Antonio Novais, 2, 28040 Madrid, Spain 3 Geosciences Institute (IGEO-UCM-CSIC), Severo Ochoa 7, 28040 Madrid, Spain 4 Federal Institute for Geosciences and Natural Resources (BGR), Stilleweg 2, D-30655 Hannover, Germany 5 HERCULES Laboratory, Évora University, Largo Marquês de Marialva, 8, 7000-809 Évora, Portugal 6 Leibniz Universität Hannover Institut für Mineralogie. Callinstraße, 3, 30167 Hannover, Germany 7 Central Laboratories, Geological Survey of Spain (IGME), C/La Calera, 1, 28760 Tres Cantos—Madrid, Spain * Correspondence: [email protected] Received: 10 May 2019; Accepted: 13 July 2019; Published: 17 July 2019 Abstract: Four pure hydrogenetic, mixed hydrogenetic-diagenetic and hydrogenetic-hydrothermal Fe-Mn Crusts from the Canary Islands Seamount Province have been studied by Micro X-Ray Diffraction, Raman and Fourier-transform infrared spectroscopy together with high resolution Electron Probe Micro Analyzer and Laser Ablation Inductively Coupled Plasma Mass Spectrometry in order to find the correlation of mineralogy and geochemistry with the three genetic processes and their influence in the metal recovery rate using an hydrometallurgical method.