The Irregular Satellites of Saturn
Total Page:16
File Type:pdf, Size:1020Kb
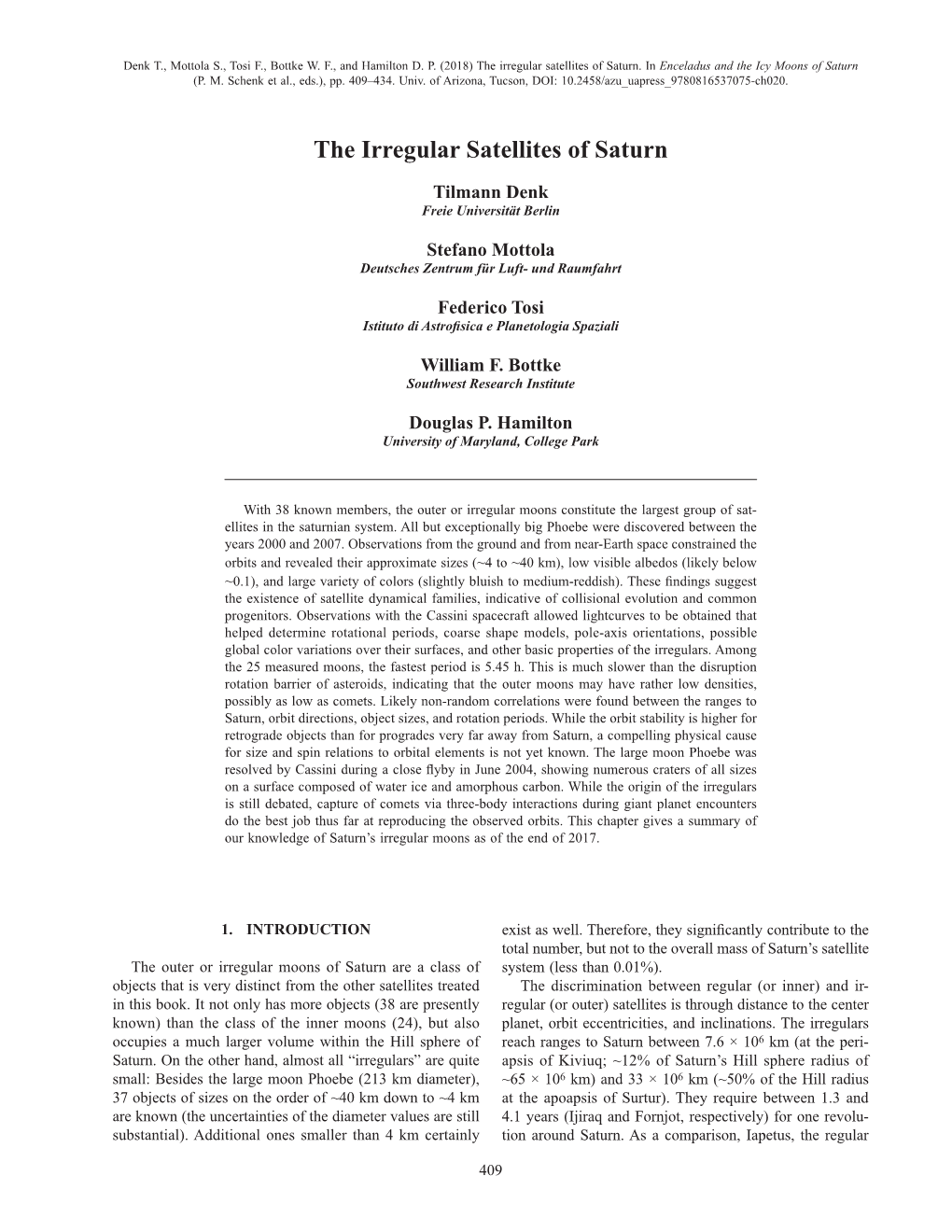
Load more
Recommended publications
-
The Geology of the Rocky Bodies Inside Enceladus, Europa, Titan, and Ganymede
49th Lunar and Planetary Science Conference 2018 (LPI Contrib. No. 2083) 2905.pdf THE GEOLOGY OF THE ROCKY BODIES INSIDE ENCELADUS, EUROPA, TITAN, AND GANYMEDE. Paul K. Byrne1, Paul V. Regensburger1, Christian Klimczak2, DelWayne R. Bohnenstiehl1, Steven A. Hauck, II3, Andrew J. Dombard4, and Douglas J. Hemingway5, 1Planetary Research Group, Department of Marine, Earth, and Atmospheric Sciences, North Carolina State University, Raleigh, NC 27695, USA ([email protected]), 2Department of Geology, University of Georgia, Athens, GA 30602, USA, 3Department of Earth, Environmental, and Planetary Sciences, Case Western Reserve University, Cleveland, OH 44106, USA, 4Department of Earth and Environmental Sciences, University of Illinois at Chicago, Chicago, IL 60607, USA, 5Department of Earth & Planetary Science, University of California Berkeley, Berkeley, CA 94720, USA. Introduction: The icy satellites of Jupiter and horizontal stresses, respectively, Pp is pore fluid Saturn have been the subjects of substantial geological pressure (found from (3)), and μ is the coefficient of study. Much of this work has focused on their outer friction [12]. Finally, because equations (4) and (5) shells [e.g., 1–3], because that is the part most readily assess failure in the brittle domain, we also considered amenable to analysis. Yet many of these satellites ductile deformation with the relation n –E/RT feature known or suspected subsurface oceans [e.g., 4– ε̇ = C1σ exp , (6) 6], likely situated atop rocky interiors [e.g., 7], and where ε̇ is strain rate, C1 is a constant, σ is deviatoric several are of considerable astrobiological significance. stress, n is the stress exponent, E is activation energy, R For example, chemical reactions at the rock–water is the universal gas constant, and T is temperature [13]. -
Water Masers in the Saturnian System
A&A 494, L1–L4 (2009) Astronomy DOI: 10.1051/0004-6361:200811186 & c ESO 2009 Astrophysics Letter to the Editor Water masers in the Saturnian system S. V. Pogrebenko1,L.I.Gurvits1, M. Elitzur2,C.B.Cosmovici3,I.M.Avruch1,4, S. Montebugnoli5 , E. Salerno5, S. Pluchino3,5, G. Maccaferri5, A. Mujunen6, J. Ritakari6, J. Wagner6,G.Molera6, and M. Uunila6 1 Joint Institute for VLBI in Europe, PO Box 2, 7990 AA Dwingeloo, The Netherlands e-mail: [pogrebenko;lgurvits]@jive.nl 2 Department of Physics and Astronomy, University of Kentucky, 600 Rose Street, Lexington, KY 40506-0055, USA e-mail: [email protected] 3 Istituto Nazionale di Astrofisica (INAF) – Istituto di Fisica dello Spazio Interplanetario (IFSI), via del Fosso del Cavaliere, 00133 Rome, Italy e-mail: [email protected] 4 Science & Technology BV, PO 608 2600 AP Delft, The Netherlands e-mail: [email protected] 5 Istituto Nazionale di Astrofisica (INAF) – Istituto di Radioastronomia (IRA) – Stazione Radioastronomica di Medicina, via Fiorentina 3508/B, 40059 Medicina (BO), Italy e-mail: [s.montebugnoli;e.salerno;g.maccaferri]@ira.inaf.it; [email protected] 6 Helsinki University of Technology TKK, Metsähovi Radio Observatory, 02540 Kylmälä, Finland e-mail: [amn;jr;jwagner;gofrito;minttu]@kurp.hut.fi Received 18 October 2008 / Accepted 4 December 2008 ABSTRACT Context. The presence of water has long been seen as a key condition for life in planetary environments. The Cassini spacecraft discovered water vapour in the Saturnian system by detecting absorption of UV emission from a background star. Investigating other possible manifestations of water is essential, one of which, provided physical conditions are suitable, is maser emission. -
Cassini Update
Cassini Update Dr. Linda Spilker Cassini Project Scientist Outer Planets Assessment Group 22 February 2017 Sols%ce Mission Inclina%on Profile equator Saturn wrt Inclination 22 February 2017 LJS-3 Year 3 Key Flybys Since Aug. 2016 OPAG T124 – Titan flyby (1584 km) • November 13, 2016 • LAST Radio Science flyby • One of only two (cf. T106) ideal bistatic observations capturing Titan’s Northern Seas • First and only bistatic observation of Punga Mare • Western Kraken Mare not explored by RSS before T125 – Titan flyby (3158 km) • November 29, 2016 • LAST Optical Remote Sensing targeted flyby • VIMS high-resolution map of the North Pole looking for variations at and around the seas and lakes. • CIRS last opportunity for vertical profile determination of gases (e.g. water, aerosols) • UVIS limb viewing opportunity at the highest spatial resolution available outside of occultations 22 February 2017 4 Interior of Hexagon Turning “Less Blue” • Bluish to golden haze results from increased production of photochemical hazes as north pole approaches summer solstice. • Hexagon acts as a barrier that prevents haze particles outside hexagon from migrating inward. • 5 Refracting Atmosphere Saturn's• 22unlit February rings appear 2017 to bend as they pass behind the planet’s darkened limb due• 6 to refraction by Saturn's upper atmosphere. (Resolution 5 km/pixel) Dione Harbors A Subsurface Ocean Researchers at the Royal Observatory of Belgium reanalyzed Cassini RSS gravity data• 7 of Dione and predict a crust 100 km thick with a global ocean 10’s of km deep. Titan’s Summer Clouds Pose a Mystery Why would clouds on Titan be visible in VIMS images, but not in ISS images? ISS ISS VIMS High, thin cirrus clouds that are optically thicker than Titan’s atmospheric haze at longer VIMS wavelengths,• 22 February but optically 2017 thinner than the haze at shorter ISS wavelengths, could be• 8 detected by VIMS while simultaneously lost in the haze to ISS. -
A Deeper Look at the Colors of the Saturnian Irregular Satellites Arxiv
A deeper look at the colors of the Saturnian irregular satellites Tommy Grav Harvard-Smithsonian Center for Astrophysics, MS51, 60 Garden St., Cambridge, MA02138 and Instiute for Astronomy, University of Hawaii, 2680 Woodlawn Dr., Honolulu, HI86822 [email protected] James Bauer Jet Propulsion Laboratory, California Institute of Technology 4800 Oak Grove Dr., MS183-501, Pasadena, CA91109 [email protected] September 13, 2018 arXiv:astro-ph/0611590v2 8 Mar 2007 Manuscript: 36 pages, with 11 figures and 5 tables. 1 Proposed running head: Colors of Saturnian irregular satellites Corresponding author: Tommy Grav MS51, 60 Garden St. Cambridge, MA02138 USA Phone: (617) 384-7689 Fax: (617) 495-7093 Email: [email protected] 2 Abstract We have performed broadband color photometry of the twelve brightest irregular satellites of Saturn with the goal of understanding their surface composition, as well as their physical relationship. We find that the satellites have a wide variety of different surface colors, from the negative spectral slopes of the two retrograde satellites S IX Phoebe (S0 = −2:5 ± 0:4) and S XXV Mundilfari (S0 = −5:0 ± 1:9) to the fairly red slope of S XXII Ijiraq (S0 = 19:5 ± 0:9). We further find that there exist a correlation between dynamical families and spectral slope, with the prograde clusters, the Gallic and Inuit, showing tight clustering in colors among most of their members. The retrograde objects are dynamically and physically more dispersed, but some internal structure is apparent. Keywords: Irregular satellites; Photometry, Satellites, Surfaces; Saturn, Satellites. 3 1 Introduction The satellites of Saturn can be divided into two distinct groups, the regular and irregular, based on their orbital characteristics. -
JUICE Red Book
ESA/SRE(2014)1 September 2014 JUICE JUpiter ICy moons Explorer Exploring the emergence of habitable worlds around gas giants Definition Study Report European Space Agency 1 This page left intentionally blank 2 Mission Description Jupiter Icy Moons Explorer Key science goals The emergence of habitable worlds around gas giants Characterise Ganymede, Europa and Callisto as planetary objects and potential habitats Explore the Jupiter system as an archetype for gas giants Payload Ten instruments Laser Altimeter Radio Science Experiment Ice Penetrating Radar Visible-Infrared Hyperspectral Imaging Spectrometer Ultraviolet Imaging Spectrograph Imaging System Magnetometer Particle Package Submillimetre Wave Instrument Radio and Plasma Wave Instrument Overall mission profile 06/2022 - Launch by Ariane-5 ECA + EVEE Cruise 01/2030 - Jupiter orbit insertion Jupiter tour Transfer to Callisto (11 months) Europa phase: 2 Europa and 3 Callisto flybys (1 month) Jupiter High Latitude Phase: 9 Callisto flybys (9 months) Transfer to Ganymede (11 months) 09/2032 – Ganymede orbit insertion Ganymede tour Elliptical and high altitude circular phases (5 months) Low altitude (500 km) circular orbit (4 months) 06/2033 – End of nominal mission Spacecraft 3-axis stabilised Power: solar panels: ~900 W HGA: ~3 m, body fixed X and Ka bands Downlink ≥ 1.4 Gbit/day High Δv capability (2700 m/s) Radiation tolerance: 50 krad at equipment level Dry mass: ~1800 kg Ground TM stations ESTRAC network Key mission drivers Radiation tolerance and technology Power budget and solar arrays challenges Mass budget Responsibilities ESA: manufacturing, launch, operations of the spacecraft and data archiving PI Teams: science payload provision, operations, and data analysis 3 Foreword The JUICE (JUpiter ICy moon Explorer) mission, selected by ESA in May 2012 to be the first large mission within the Cosmic Vision Program 2015–2025, will provide the most comprehensive exploration to date of the Jovian system in all its complexity, with particular emphasis on Ganymede as a planetary body and potential habitat. -
“Savage and Deformed”: Stigma As Drama in the Tempest Jeffrey R
“Savage and Deformed”: Stigma as Drama in The Tempest Jeffrey R. Wilson The dramatis personae of The Tempest casts Caliban as “asavageand deformed slave.”1 Since the mid-twentieth century, critics have scrutinized Caliban’s status as a “slave,” developing a riveting post-colonial reading of the play, but I want to address the pairing of “savage and deformed.”2 If not Shakespeare’s own mixture of moral and corporeal abominations, “savage and deformed” is the first editorial comment on Caliban, the “and” here Stigmatized as such, Caliban’s body never comes to us .”ס“ working as an uninterpreted. It is always already laden with meaning. But what, if we try to strip away meaning from fact, does Caliban actually look like? The ambiguous and therefore amorphous nature of Caliban’s deformity has been a perennial problem in both dramaturgical and critical studies of The Tempest at least since George Steevens’s edition of the play (1793), acutely since Alden and Virginia Vaughan’s Shakespeare’s Caliban: A Cultural His- tory (1993), and enduringly in recent readings by Paul Franssen, Julia Lup- ton, and Mark Burnett.3 Of all the “deformed” images that actors, artists, and critics have assigned to Caliban, four stand out as the most popular: the devil, the monster, the humanoid, and the racial other. First, thanks to Prospero’s yarn of a “demi-devil” (5.1.272) or a “born devil” (4.1.188) that was “got by the devil himself” (1.2.319), early critics like John Dryden and Joseph War- ton envisioned a demonic Caliban.4 In a second set of images, the reverbera- tions of “monster” in The Tempest have led writers and artists to envision Caliban as one of three prodigies: an earth creature, a fish-like thing, or an animal-headed man. -
Trans-Neptunian Objects a Brief History, Dynamical Structure, and Characteristics of Its Inhabitants
Trans-Neptunian Objects A Brief history, Dynamical Structure, and Characteristics of its Inhabitants John Stansberry Space Telescope Science Institute IAC Winter School 2016 IAC Winter School 2016 -- Kuiper Belt Overview -- J. Stansberry 1 The Solar System beyond Neptune: History • 1930: Pluto discovered – Photographic survey for Planet X • Directed by Percival Lowell (Lowell Observatory, Flagstaff, Arizona) • Efforts from 1905 – 1929 were fruitless • discovered by Clyde Tombaugh, Feb. 1930 (33 cm refractor) – Survey continued into 1943 • Kuiper, or Edgeworth-Kuiper, Belt? – 1950’s: Pluto represented (K.E.), or had scattered (G.K.) a primordial, population of small bodies – thus KBOs or EKBOs – J. Fernandez (1980, MNRAS 192) did pretty clearly predict something similar to the trans-Neptunian belt of objects – Trans-Neptunian Objects (TNOs), trans-Neptunian region best? – See http://www2.ess.ucla.edu/~jewitt/kb/gerard.html IAC Winter School 2016 -- Kuiper Belt Overview -- J. Stansberry 2 The Solar System beyond Neptune: History • 1978: Pluto’s moon, Charon, discovered – Photographic astrometry survey • 61” (155 cm) reflector • James Christy (Naval Observatory, Flagstaff) – Technologically, discovery was possible decades earlier • Saturation of Pluto images masked the presence of Charon • 1988: Discovery of Pluto’s atmosphere – Stellar occultation • Kuiper airborne observatory (KAO: 90 cm) + 7 sites • Measured atmospheric refractivity vs. height • Spectroscopy suggested N2 would dominate P(z), T(z) • 1992: Pluto’s orbit explained • Outward migration by Neptune results in capture into 3:2 resonance • Pluto’s inclination implies Neptune migrated outward ~5 AU IAC Winter School 2016 -- Kuiper Belt Overview -- J. Stansberry 3 The Solar System beyond Neptune: History • 1992: Discovery of 2nd TNO • 1976 – 92: Multiple dedicated surveys for small (mV > 20) TNOs • Fall 1992: Jewitt and Luu discover 1992 QB1 – Orbit confirmed as ~circular, trans-Neptunian in 1993 • 1993 – 4: 5 more TNOs discovered • c. -
Privacy Policy
Privacy Policy Culmia Privacy Policy Culmia Desarrollos Inmobiliarios, S.L.U. (hereinafter, “the manager”), with headquarters in Madrid, Calle Génova, 27, Company Tax No. B-67186999, is an integral manager of property assets and a member of a Property Group to which it provides its services. The manager provides its services to the following property developers who are members of its Group: Company Company Company Company Tax Tax No. No. Saturn Holdco S.A.U. A88554100 Antea Activos Inmobiliarios B88554324 S.L.U. Thrym Activos Inmobiliarios S.L.U. B88554142 Redes Promotora 1 Ma, B88093117 S.L.U. Erriap Activos Inmobiliarios S.L.U. B88554159 Redes Promotora 2 Ma, B88093174 S.L.U. Daphne Activos Inmobiliarios S.L.U. B88554167 Redes Promotora 3 Ma, B88093265 S.L.U. Dione Activos Inmobiliarios S.L.U. B88554183 Redes Promotora 4 Ma, B88093356 S.L.U. Fornjot Activos Inmobiliarios S.L.U. B88554191 Redes Promotora 5 Ma, B88093471 S.L.U. Greip Activos Inmobiliarios S.L.U. B88554217 Redes Promotora 6 Ma, B88145073 S.L.U. Hiperion Activos Inmobiliarios B88554233 Redes Promotora 7 Ma, B88145123 S.L.U. S.L.U. Loge Activos Inmobiliarios S.L.U. B88554241 Redes Promotora 8 Ma, B88145263 S.L.U. Kiviuq Activos Inmobiliarios S.L.U. B88554258 Redes Promotora 9, S.L.U. B88159728 Narvi Activos Inmobiliarios S.L.U. B88554266 Redes 2 2018 Iberica 2 B88160221 S.L.U. Polux Activos Inmobiliarios S.L.U. B88554282 Redes 2 2018 Iberica 3 B88160239 S.L.U. Siarnaq Activos Inmobiliarios S.L.U. B88554290 Redes 2 Promotora B88160247 Inversiones 2018 IV S.L.U. -
The Minor Planet Bulletin
THE MINOR PLANET BULLETIN OF THE MINOR PLANETS SECTION OF THE BULLETIN ASSOCIATION OF LUNAR AND PLANETARY OBSERVERS VOLUME 36, NUMBER 3, A.D. 2009 JULY-SEPTEMBER 77. PHOTOMETRIC MEASUREMENTS OF 343 OSTARA Our data can be obtained from http://www.uwec.edu/physics/ AND OTHER ASTEROIDS AT HOBBS OBSERVATORY asteroid/. Lyle Ford, George Stecher, Kayla Lorenzen, and Cole Cook Acknowledgements Department of Physics and Astronomy University of Wisconsin-Eau Claire We thank the Theodore Dunham Fund for Astrophysics, the Eau Claire, WI 54702-4004 National Science Foundation (award number 0519006), the [email protected] University of Wisconsin-Eau Claire Office of Research and Sponsored Programs, and the University of Wisconsin-Eau Claire (Received: 2009 Feb 11) Blugold Fellow and McNair programs for financial support. References We observed 343 Ostara on 2008 October 4 and obtained R and V standard magnitudes. The period was Binzel, R.P. (1987). “A Photoelectric Survey of 130 Asteroids”, found to be significantly greater than the previously Icarus 72, 135-208. reported value of 6.42 hours. Measurements of 2660 Wasserman and (17010) 1999 CQ72 made on 2008 Stecher, G.J., Ford, L.A., and Elbert, J.D. (1999). “Equipping a March 25 are also reported. 0.6 Meter Alt-Azimuth Telescope for Photometry”, IAPPP Comm, 76, 68-74. We made R band and V band photometric measurements of 343 Warner, B.D. (2006). A Practical Guide to Lightcurve Photometry Ostara on 2008 October 4 using the 0.6 m “Air Force” Telescope and Analysis. Springer, New York, NY. located at Hobbs Observatory (MPC code 750) near Fall Creek, Wisconsin. -
PACS Sky Fields and Double Sources for Photometer Spatial Calibration
Document: PACS-ME-TN-035 PACS Date: 27th July 2009 Herschel Version: 2.7 Fields and Double Sources for Spatial Calibration Page 1 PACS Sky Fields and Double Sources for Photometer Spatial Calibration M. Nielbock1, D. Lutz2, B. Ali3, T. M¨uller2, U. Klaas1 1Max{Planck{Institut f¨urAstronomie, K¨onigstuhl17, D-69117 Heidelberg, Germany 2Max{Planck{Institut f¨urExtraterrestrische Physik, Giessenbachstraße, D-85748 Garching, Germany 3NHSC, IPAC, California Institute of Technology, Pasadena, CA 91125, USA Document: PACS-ME-TN-035 PACS Date: 27th July 2009 Herschel Version: 2.7 Fields and Double Sources for Spatial Calibration Page 2 Contents 1 Scope and Assumptions 4 2 Applicable and Reference Documents 4 3 Stars 4 3.1 Optical Star Clusters . .4 3.2 Bright Binaries (V -band search) . .5 3.3 Bright Binaries (K-band search) . .5 3.4 Retrieval from PACS Pointing Calibration Target List . .5 3.5 Other stellar sources . 13 3.5.1 Herbig Ae/Be stars observed with ISOPHOT . 13 4 Galactic ISOCAM fields 13 5 Galaxies 13 5.1 Quasars and AGN from the Veron catalogue . 13 5.2 Galaxy pairs . 14 5.2.1 Galaxy pairs from the IRAS Bright Galaxy Sample with VLA radio observations 14 6 Solar system objects 18 6.1 Asteroid conjunctions . 18 6.2 Conjunctions of asteroids with pointing stars . 22 6.3 Planetary satellites . 24 Appendices 26 A 2MASS images of fields with suitable double stars from the K-band 26 B HIRES/2MASS overlays for double stars from the K-band search 32 C FIR/NIR overlays for double galaxies 38 C.1 HIRES/2MASS overlays for double galaxies . -
Cassini Observations of Saturn's Irregular Moons
EPSC Abstracts Vol. 12, EPSC2018-103-1, 2018 European Planetary Science Congress 2018 EEuropeaPn PlanetarSy Science CCongress c Author(s) 2018 Cassini Observations of Saturn's Irregular Moons Tilmann Denk (1) and Stefano Mottola (2) (1) Freie Universität Berlin, Germany ([email protected]), (2) DLR Berlin, Germany 1. Introduction two prograde irregulars are slower than ~13 h, while the periods of all but two retrogrades are faster than With the ISS-NAC camera of the Cassini spacecraft, ~13 h. The fastest period (Hati) is much slower than we obtained photometric lightcurves of 25 irregular the disruption rotation barrier for asteroids (~2.3 h), moons of Saturn. The goal was to derive basic phys- indicating that Saturn's irregulars may be rubble piles ical properties of these objects (like rotational periods, of rather low densities, possibly as low as of comets. shapes, pole-axis orientations, possible global color variations, ...) and to get hints on their formation and Table: Rotational periods of 25 Saturnian irregulars evolution. Our campaign marks the first utilization of an interplanetary probe for a systematic photometric Moon Approx. size Rotational period survey of irregular moons. name [km] [h] Hati 5 5.45 ± 0.04 The irregular moons are a class of objects that is very Mundilfari 7 6.74 ± 0.08 distinct from the inner moons of Saturn. Not only are Loge 5 6.9 ± 0.1 ? they more numerous (38 versus 24), but also occupy Skoll 5 7.26 ± 0.09 (?) a much larger volume within the Hill sphere of Suttungr 7 7.67 ± 0.02 Saturn. -
Dunham Discuss May 30 Eclipse Results
c * Volume XLI November 1984 Number 3 DUNHAM TO DISCUSS MAY 30 ECLIPSE RESULTS Dr. David W. Dunham, President of the International Occultation Timing Association (IOTA), will address the November meeting of National Capital Astronome r s on small change s in the radius of the Sun derived from solar eclipse observations. In 1979, astronomers at Harvard University startled the scientific community with the news that the Sun was shrinking, based on their analy- sis of two centuries of meridian-circle obser- vations of the Sun. The Dunhams analyzed their timings, and those of others who observed just inside the 1979 February 26 eclipse, to determine the solar diameter, and compared this with a similar analysis of eclipses observed in 1915 and 1925. Although the large shrinkage of the Sun was disproved, small variations of the radius were found, which puzzled solar physicists. The Dunhams will describe the methods of analysis and results from recent eclipses. The May 30 eclipse provided an unusual opportunity to re cord Bailey's beads with a variety of methods to determine which techniques are best for measuring the Sun. The Dunharn s ' video recording of the eclipse as well as some observations by IOTA and NCA members will be shown. Dr. Joan Dunham collaborates. David W. Dunham received his B.A. in astronomy from the University of California, Berkeley, in 1964, and his Ph.D. in celestial mechanics from Yale in 1971. He joined Computer Science Corporation in 1976, where he is involved in dynamic analyses of space missions. Since 1964 he has worked closely with the U.