JAERI-Review 2001-040 REVIEW of ACTINIDE NITRIDE PROPERTIES
Total Page:16
File Type:pdf, Size:1020Kb
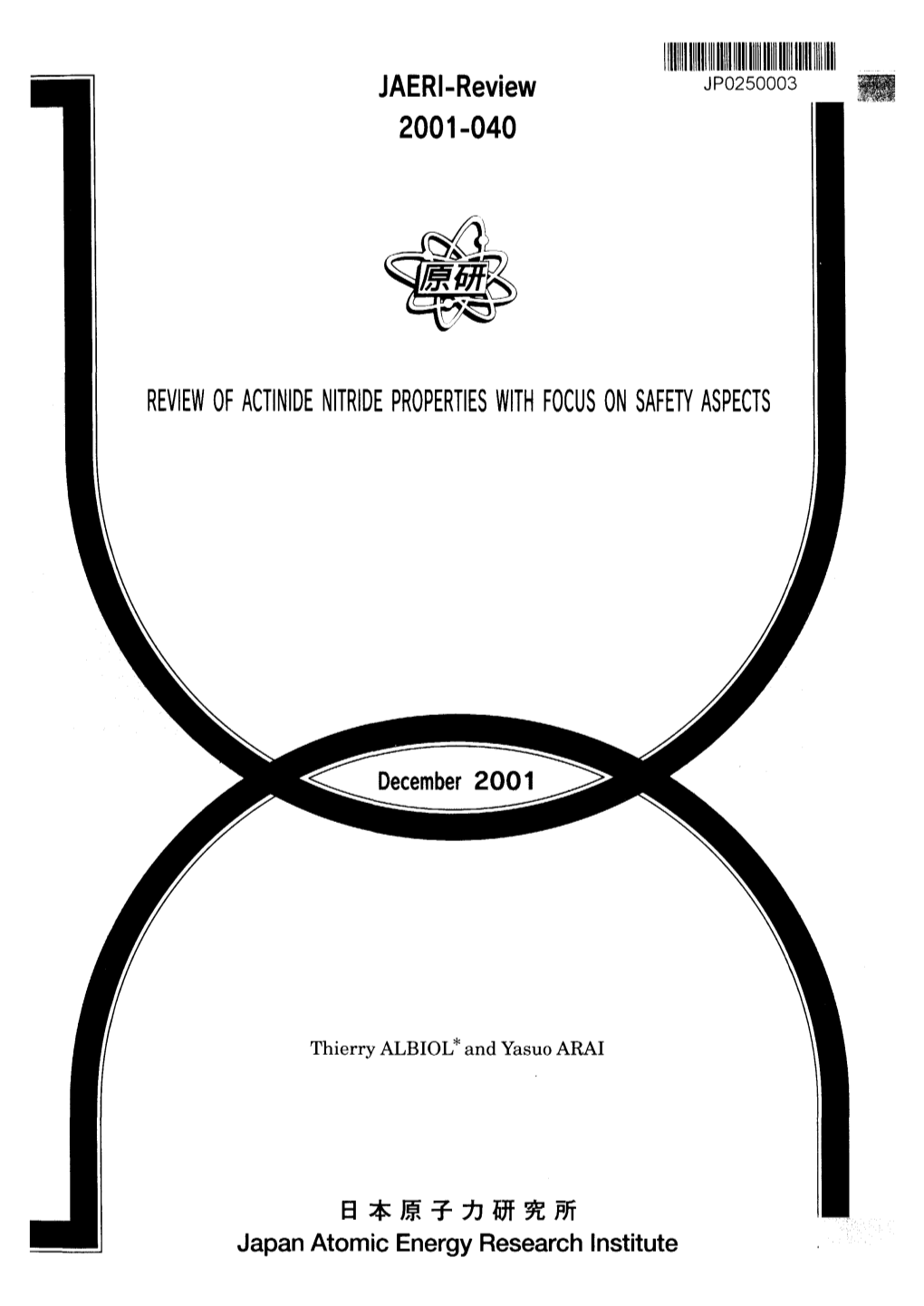
Load more
Recommended publications
-
Transport of Dangerous Goods
ST/SG/AC.10/1/Rev.16 (Vol.I) Recommendations on the TRANSPORT OF DANGEROUS GOODS Model Regulations Volume I Sixteenth revised edition UNITED NATIONS New York and Geneva, 2009 NOTE The designations employed and the presentation of the material in this publication do not imply the expression of any opinion whatsoever on the part of the Secretariat of the United Nations concerning the legal status of any country, territory, city or area, or of its authorities, or concerning the delimitation of its frontiers or boundaries. ST/SG/AC.10/1/Rev.16 (Vol.I) Copyright © United Nations, 2009 All rights reserved. No part of this publication may, for sales purposes, be reproduced, stored in a retrieval system or transmitted in any form or by any means, electronic, electrostatic, magnetic tape, mechanical, photocopying or otherwise, without prior permission in writing from the United Nations. UNITED NATIONS Sales No. E.09.VIII.2 ISBN 978-92-1-139136-7 (complete set of two volumes) ISSN 1014-5753 Volumes I and II not to be sold separately FOREWORD The Recommendations on the Transport of Dangerous Goods are addressed to governments and to the international organizations concerned with safety in the transport of dangerous goods. The first version, prepared by the United Nations Economic and Social Council's Committee of Experts on the Transport of Dangerous Goods, was published in 1956 (ST/ECA/43-E/CN.2/170). In response to developments in technology and the changing needs of users, they have been regularly amended and updated at succeeding sessions of the Committee of Experts pursuant to Resolution 645 G (XXIII) of 26 April 1957 of the Economic and Social Council and subsequent resolutions. -
Uranium Pyrophoricity Phenomena and Prediction
SNF-6192-FP URANIUM PYROPHORICITY PHENOMENA AND PREDICTION Martin G. Plys, Michael Epstein, and Boro Malinovic Fauske & Associates, Inc. 16W070 W. 83rd St. Burr Ridge, Illinois 60521 USA (630) 323-8750 [email protected] ABSTRACT We have compiled a topical reference on the phenomena, experiences, experiments, and prediction of uranium pyrophoricity for the Hanford Spent Nuclear Fuel Project (SNFP) with specific applications to SNFP process and situations. The purpose of the compilation is to create a reference to integrate and preserve this knowledge. Decades ago, uranium and zirconium fires were commonplace at Atomic Energy Commission facilities, and good documentation of experiences is surprisingly sparse. Today, these phenomena are important to site remediation and analysis of packaging, transportation, and processing of unirradiated metal scrap and spent nuclear fuel. Our document, bearing the same title as this paper, will soon be available in the Hanford document system [Plys, et al., 2000]. This paper explains general content of our topical reference and provides examples useful throughout the DOE complex. Moreover, the methods described here can be applied to analysis of potentially pyrophoric plutonium, metal, or metal hydride compounds provided that kinetic data are available. A key feature of this paper is a set of straightforward equations and values that are immediately applicable to safety analysis. 1.0 BACKGROUND AND PURPOSE The phenomenon of pyrophoricity has been studied for chemical process safety and its mathematical formulation, ignition theory, is well established. We have applied ignition theory to experiments conducted with uranium powders and foils using recently available kinetic rate laws, and found that results can be explained and understood, where before these results were not quantified and were on occasion misinterpreted [Epstein, et al., 1996]. -
Reactions of Lithium Nitride with Some Unsaturated Organic Compounds. Perry S
Louisiana State University LSU Digital Commons LSU Historical Dissertations and Theses Graduate School 1963 Reactions of Lithium Nitride With Some Unsaturated Organic Compounds. Perry S. Mason Jr Louisiana State University and Agricultural & Mechanical College Follow this and additional works at: https://digitalcommons.lsu.edu/gradschool_disstheses Recommended Citation Mason, Perry S. Jr, "Reactions of Lithium Nitride With Some Unsaturated Organic Compounds." (1963). LSU Historical Dissertations and Theses. 898. https://digitalcommons.lsu.edu/gradschool_disstheses/898 This Dissertation is brought to you for free and open access by the Graduate School at LSU Digital Commons. It has been accepted for inclusion in LSU Historical Dissertations and Theses by an authorized administrator of LSU Digital Commons. For more information, please contact [email protected]. This dissertation has been 64—5058 microfilmed exactly as received MASON, Jr., Perry S., 1938- REACTIONS OF LITHIUM NITRIDE WITH SOME UNSATURATED ORGANIC COMPOUNDS. Louisiana State University, Ph.D., 1963 Chemistry, organic University Microfilms, Inc., Ann Arbor, Michigan Reproduced with permission of the copyright owner. Further reproduction prohibited without permission. Reproduced with permission of the copyright owner. Further reproduction prohibited without permission. Reproduced with permission of the copyright owner. Further reproduction prohibited without permission. REACTIONS OF LITHIUM NITRIDE WITH SOME UNSATURATED ORGANIC COMPOUNDS A Dissertation Submitted to the Graduate Faculty of the Louisiana State University and Agricultural and Mechanical College in partial fulfillment of the requireiaents for the degree of Doctor of Philosophy in The Department of Chemistry by Perry S. Mason, Jr. B. S., Harding College, 1959 August, 1963 Reproduced with permission of the copyright owner. Further reproduction prohibited without permission. -
High-Quality, Low-Cost Bulk Gallium Nitride Substrates
ADVANCED MANUFACTURING OFFICE High-Quality, Low- Cost Bulk Gallium Nitride Substrates Electrochemical Solution Growth: A Scalable Semiconductor Manufacturing Process The ever-growing demand in the past decade for more energy efficient solid-state lighting and electrical power conversion is leading to a higher demand for wide bandgap semiconductor-based devices, such as gallium nitride (GaN), over traditional silicon (Si)-based devices. High cost and limited availability, how- ever, have hindered the adoption of GaN substrates to date. When utilizing GaN, current LED and power electronic device applications employ GaN epitaxially grown on top of non-GaN substrates. The lattice mismatch between the epitaxial GaN layer and the non-native substrate surface leads to con- siderable stress and high defect densities, ultimately compromising device yield and Conceptual diagram of the ESG reactor. Photo courtesy of Sandia National Laboratories performance. While bulk growth of GaN can combat these issues, current growth methods for bulk GaN have not fostered widespread adoption to date due to lim- This project will help develop ESG into a viable GaN bulk growth process that is well ited scalability, low material quality, high suited for scalability to large-area wafer manufacturing. Bulk GaN is important to bol- operating temperatures and pressures, stering U.S. competitiveness in high-efficiency power electronics and solid-state lighting. and slow growth rates. A fundamentally different manufacturing route for bulk Benefits for Our Industry and Our Nation growth of GaN not driven by thermal pro- Scaling the ESG growth method to large area GaN crystals could reduce the production cesses is needed to provide an adequate cost of bulk GaN wafers by up to a factor of 10. -
Transition-Metal-Bridged Bimetallic Clusters with Multiple Uranium–Metal Bonds
ARTICLES https://doi.org/10.1038/s41557-018-0195-4 Transition-metal-bridged bimetallic clusters with multiple uranium–metal bonds Genfeng Feng1, Mingxing Zhang1, Dong Shao 1, Xinyi Wang1, Shuao Wang2, Laurent Maron 3* and Congqing Zhu 1* Heterometallic clusters are important in catalysis and small-molecule activation because of the multimetallic synergistic effects from different metals. However, multimetallic species that contain uranium–metal bonds remain very scarce due to the difficulties in their synthesis. Here we present a straightforward strategy to construct a series of heterometallic clusters with multiple uranium–metal bonds. These complexes were created by facile reactions of a uranium precursor with Ni(COD)2 (COD, cyclooctadiene). The multimetallic clusters’ cores are supported by a heptadentate N4P3 scaffold. Theoretical investigations indicate the formation of uranium–nickel bonds in a U2Ni2 and a U2Ni3 species, but also show that they exhibit a uranium–ura- nium interaction; thus, the electronic configuration of uranium in these species is U(III)-5f26d1. This study provides further understanding of the bonding between f-block elements and transition metals, which may allow the construction of d–f hetero- metallic clusters and the investigation of their potential applications. ultimetallic molecules are of great interest because of This study offers a new opportunity to investigate d− f heteromul- their fascinating structures and multimetallic synergistic timetallic clusters with multiple uranium–metal bonds for small- Meffects for catalysis and small molecule activation1–7. Both molecule activation and catalysis. biological nitrogen fixation and industrial Haber–Bosch ammonia syntheses, for example, are thought to utilize multimetallic cata- Results and discussion lytic sites8,9. -
Naming Compounds Practice Problems KEY Naming Simple Ionic Compounds
Chemistry HS/Science Unit: 05 Lesson: 01 Naming Compounds Practice Problems KEY Naming Simple Ionic Compounds Name the following compounds: 1) KCl potassium chloride 2) MgI2 magnesium iodide 3) FeO iron (II) oxide 4) Fe2O3 iron (III) oxide 5) Cu3P copper (I) phosphide 6) SnSe2 tin (IV) selenide 7) TiBr3 titanium (III) bromide 8) GaAs gallium arsenide 9) BeF2 beryllium fluoride 10) Cs3N cesium nitride Write the formulas for the following compounds: 1) lithium iodide LiI 2) cobalt (III) oxide Co2O3 3) calcium fluoride CaF2 4) silver bromide AgBr 5) sodium hydride NaH 6) vanadium (V) sulfide V2S5 7) lead (II) nitride Pb3N2 8) titanium (II) selenide TiSe 9) manganese (VII) arsenide Mn3As7 10) gallium chloride GaCl3 ©2013, TESCCC 06/17/13 page 1 of 4 Chemistry HS/Science Unit: 05 Lesson: 01 Naming Compounds Practice Problems Naming Complex (polyatomic) Ionic Compounds Name the following compounds: 1) NH4Cl ammonium chloride 2) Fe(NO3)3 iron (III) nitrate 3) Pb(SO4)2 lead (IV) sulfate 4) Ag3PO4 silver phosphate 5) Be(HCO3)2 beryllium hydrogen carbonate 6) Al(CN)3 aluminum cyanide 7) Mn2(SO3)3 manganese (III) sulfite 8) Sr(C2H3O2)2 strontium acetate 9) Ti(CN)4 titanium (IV) cyanide 10) YClO3 yttrium chlorate Write the formulas for the following compounds: 1) lead (IV) sulfate Pb(SO4)2 2) silver cyanide AgCN 3) copper (II) chlorate Cu(ClO3)2 4) chromium (IV) phosphate Cr3(PO4)4 5) vanadium (IV) carbonate V(CO3)2 6) ammonium oxide (NH4)2O 7) tin (II) nitrite Sn(NO2)2 8) chromium (III) hydroxide Cr(OH)3 9) titanium (II) acetate Ti(C2H3O2)2 10) -
Rational Ligand Design for U(VI) and Pu(IV)* by Géza Szigethy BA
Rational Ligand Design for U(VI) and Pu(IV)* by Géza Szigethy B.A. (Princeton University), 2004 A dissertation submitted in partial satisfaction of the requirements for the degree of Doctor of Philosophy in Chemistry in the Graduate Division of the University of California, Berkeley Committee in charge: Professor Kenneth N. Raymond, Chair Professor Richard A. Andersen Professor Garrison Sposito Fall 2009 * This research and the ALS are supported by the Director, Office of Science, Office of Basic Energy Sciences (OBES), and the OBES Division of Chemical Sciences, Geosciences, and Biosciences of the U.S. Department of Energy at LBNL under Contract No. DE-AC02- 05CH11231. Rational Ligand Design for U(VI) and Pu(IV) by Géza Szigethy B.A. (Princeton University), 2004 A dissertation submitted in partial satisfaction of the requirements for the degree of Doctor of Philosophy in Chemistry in the Graduate Division of the University of California, Berkeley Committee in charge: Professor Kenneth N. Raymond, Chair Professor Richard A. Andersen Professor Garrison Sposito Fall 2009 Rational Ligand Design for U(VI) and Pu(IV) Copyright © 2009 Géza Szigethy Abstract Rational Ligand Design for U(VI) and Pu(IV) by Géza Szigethy Doctor of Philosophy in Chemistry University of California, Berkeley Professor Kenneth N. Raymond, Chair Nuclear power is an attractive alternative to hydrocarbon-based energy production at a time when moving away from carbon-producing processes is widely accepted as a significant developmental need. Hence, the radioactive actinide power sources for this industry are necessarily becoming more widespread, which is accompanied by the increased risk of exposure to both biological and environmental systems. -
MICROREVIEW Reactivity of Polar Organometallic Compounds In
MICROREVIEW Reactivity of Polar Organometallic Compounds in Unconventional Reaction Media: Challenges and Opportunities Joaquin García-Álvarez,*[a] Eva Hevia,*[b] and Vito Capriati*[c] This paper is gratefully dedicated to the memory of Dr. Guy Lavigne Abstract: Developing new green solvents in designing chemical chemicals in chemical transformations is, indeed, solvents used products and processes or successfully employing the already as reaction media, which account for 80–90% of mass utilization existing ones is one of the key subjects in Green Chemistry and is in a typical pharmaceutical/fine chemical operational process. especially important in Organometallic Chemistry, which is an Thus, the solvent itself is often a critical parameter especially in interdisciplinary field. Can we advantageously use unconventional drug product manufacturing and is as well responsible for most reaction media in place of current harsh organic solvents also for waste generated in the chemical industries and laboratories.[3] polar organometallic compounds? This Microreview critically Following these considerations, some of the most critical analyses the state-of-the-art on this topic and showcases recent and intriguing questions that arise are: Can we get traditional developments and breakthroughs which are becoming new research organic solvents out of organometallic reactions?[4] Can we use directions in this field. Because metals cover a vast swath of the protic, recyclable, biodegradable, and cheap unconventional periodic table, the content is organised into three Sections solvents also for highly reactive organometallic compounds? discussing the reactivity of organometallic compounds of s-, p- and Answering these questions would not only mean to break new d-block elements in unconventional solvents. -
Chelation of Actinides
UC Berkeley UC Berkeley Previously Published Works Title Chelation of Actinides Permalink https://escholarship.org/uc/item/4b57t174 Author Abergel, RJ Publication Date 2017 DOI 10.1039/9781782623892-00183 Peer reviewed eScholarship.org Powered by the California Digital Library University of California Chapter 6 Chelation of Actinides rebecca J. abergela aChemical Sciences Division, lawrence berkeley National laboratory, One Cyclotron road, berkeley, Ca 94720, USa *e-mail: [email protected] 6.1 The Medical and Public Health Relevance of Actinide Chelation the use of actinides in the civilian industry and defense sectors over the past 60 years has resulted in persistent environmental and health issues, since a large inventory of radionuclides, including actinides such as thorium (th), uranium (U), neptunium (Np), plutonium (pu), americium (am) and curium 1 Downloaded by Lawrence Berkeley National Laboratory on 22/06/2018 20:28:11. (Cm), are generated and released during these activities. Controlled process- Published on 18 October 2016 http://pubs.rsc.org | doi:10.1039/9781782623892-00183 ing and disposal of wastes from the nuclear fuel cycle are the main source of actinide dissemination. however, significant quantities of these radionu- clides have also been dispersed as a consequence of nuclear weapons testing, nuclear power plant accidents, and compromised storage of nuclear materi- als.1 In addition, events of the last fifteen years have heightened public con- cern that actinides may be released as the result of the potential terrorist use of radiological dispersal devices or after a natural disaster affecting nuclear power plants or nuclear material storage sites.2,3 all isotopes of the 15 ele- ments of the actinide series (atomic numbers 89 through 103, Figure 6.1) are radioactive and have the potential to be harmful; the heaviest members, however, are too unstable to be isolated in quantities larger than a few atoms at a time,4 and those elements cited above (U, Np, pu, am, Cm) are the most RSC Metallobiology Series No. -
Safe Handling of Organolithium Compounds in the Laboratory
FEATURE Safe handling of organolithium compounds in the laboratory Organolithium compounds are extremely useful reagents in organic synthesis and as initiators in anionic polymerizations. These reagents are corrosive, flammable, and in certain cases, pyrophoric. Careful planning prior to execution of the experiment will minimize hazards to personnel and the physical plant. The proper personal protective equipment (PPE) for handling organolithium compounds will be identified. Procedures to minimize contact with air and moisture will be presented. Solutions of organolithium compounds can be safely transferred from the storage bottles to the reaction flask with either a syringe or a cannula. With the utilization of these basic techniques, organolithium compounds can be safely handled in the laboratory. By James A. Schwindeman, oxides (typi®ed by lithium t-butoxide). various classes of organolithium com- Chris J. Woltermann, and These organolithium compounds have pounds, with pKa from 15.2 (lithium Robert J. Letchford found wide utility as reagents for methoxide) to 53 (t-butyllithium).5 organic synthesis in a variety of appli- Fourth, organolithium reagents demon- cations. For example, they can be strate enhanced nucleophilicity com- INTRODUCTION employed as strong bases (alkyl- pared to the corresponding organo- When properly handled, organo- lithiums, aryllithiums, lithium amides magnesium compound. Finally, they lithiums provide unique properties and lithium alkoxides), nucleophiles are convenient, as a variety of organo- that allow for -
NFPA 484 Standard for Combustible Metals, Metal Powders, and Metal
NFPA 484 Standard for Combustible Metals, Metal Powders, and Metal Dusts 2002 Edition NFPA, 1 Batterymarch Park, PO Box 9101, Quincy, MA 02269-9101 An International Codes and Standards Organization NFPA License Agreement This document is copyrighted by the National Fire Protection Association (NFPA), 1 Batterymarch Park, Quincy, MA 02269-9101 USA. All rights reserved. NFPA grants you a license as follows: The right to download an electronic file of this NFPA document for temporary storage on one computer for purposes of viewing and/or printing one copy of the NFPA document for individual use. Neither the electronic file nor the hard copy print may be reproduced in any way. In addition, the electronic file may not be distributed elsewhere over computer networks or otherwise. The hard copy print may only be used personally or distributed to other employees for their internal use within your organization. Copyright National Fire Protection Association, Inc. One Batterymarch Park Quincy, Massachusetts 02269 IMPORTANT NOTICE ABOUT THIS DOCUMENT NFPA codes, standards, recommended practices, and guides, of which the document contained herein is one, are developed through a consensus standards development process approved by the American National Standards Institute. This process brings together volunteers representing varied viewpoints and interests to achieve consensus on fire and other safety issues. While the NFPA administers the process and establishes rules to promote fairness in the development of consensus, it does not independently test, evaluate, or verify the accuracy of any information or the soundness of any judgments contained in its codes and standards. The NFPA disclaims liability for any personal injury, property or other damages of any nature whatsoever, whether special, indirect, consequential or compensatory, directly or indirectly resulting from the publication, use of, or reliance on this document. -
Boron-Nitride-Datasheet-V1
A Unique Proposition for Industry The introduction of boron nitride into our stable of 2D materials widens the focus of delivering innovative solutions for industry Neill Ricketts, CEO Our latest 2D success story Hexotene is a few-layer hexagonal boron nitride (h-BN) nanoplatelet powder with large lateral dimensions. With high chemical purity and mono-layer particles confirmed, Hexotene is the latest addition to our high performance 2D product range. It’s unique characteristics, specifically with regards to electrical conductivity, show some markedly different properties when compared to graphene. This is particularly promising for combined projects using both graphene and boron nitride. Example BN Nano platelets Information Property Measurement Method Boron 42 è 2.0At.% Predominantly few-layer with some Raman Nitrogen 45 è 2.0At.% Layers mono-layer and bi-layer spectroscopy Oxygen 3.0 è 1.0At.% Lateral Carbon 8.0 è 2.0At.% up to 5.0 µm SEM dimensions Method XPS IR UV Wavelenght (nm) 4096 1024 256 Band Gap ~6eV Energy BORON NITRIDE GRAPHENE Graphene Metals Semi- Plastics Boron Conductor Nitride 0.125 0.25 0.50 1.00 2.00 4.00 8.00 Conductors Semi-Conductors Insulators Energy (eV) Diagram showing extremes of electrical conductivity, with Hexagonal boron nitride is white in colour and is unusual as boron nitride having an ultra-wide bandgap. it absorbs high energy UV light, whereas graphene absorbs all light frequencies. What is h-BN? Boron and nitrogen are neighbours of carbon in boron nitride (h-BN). It also happens to be the the Periodic Table. Just as carbon can exist as softest of the BN polymorphs.