Chelidonichthys Capensis) and the Lesser Gurnard (C. Queketti
Total Page:16
File Type:pdf, Size:1020Kb
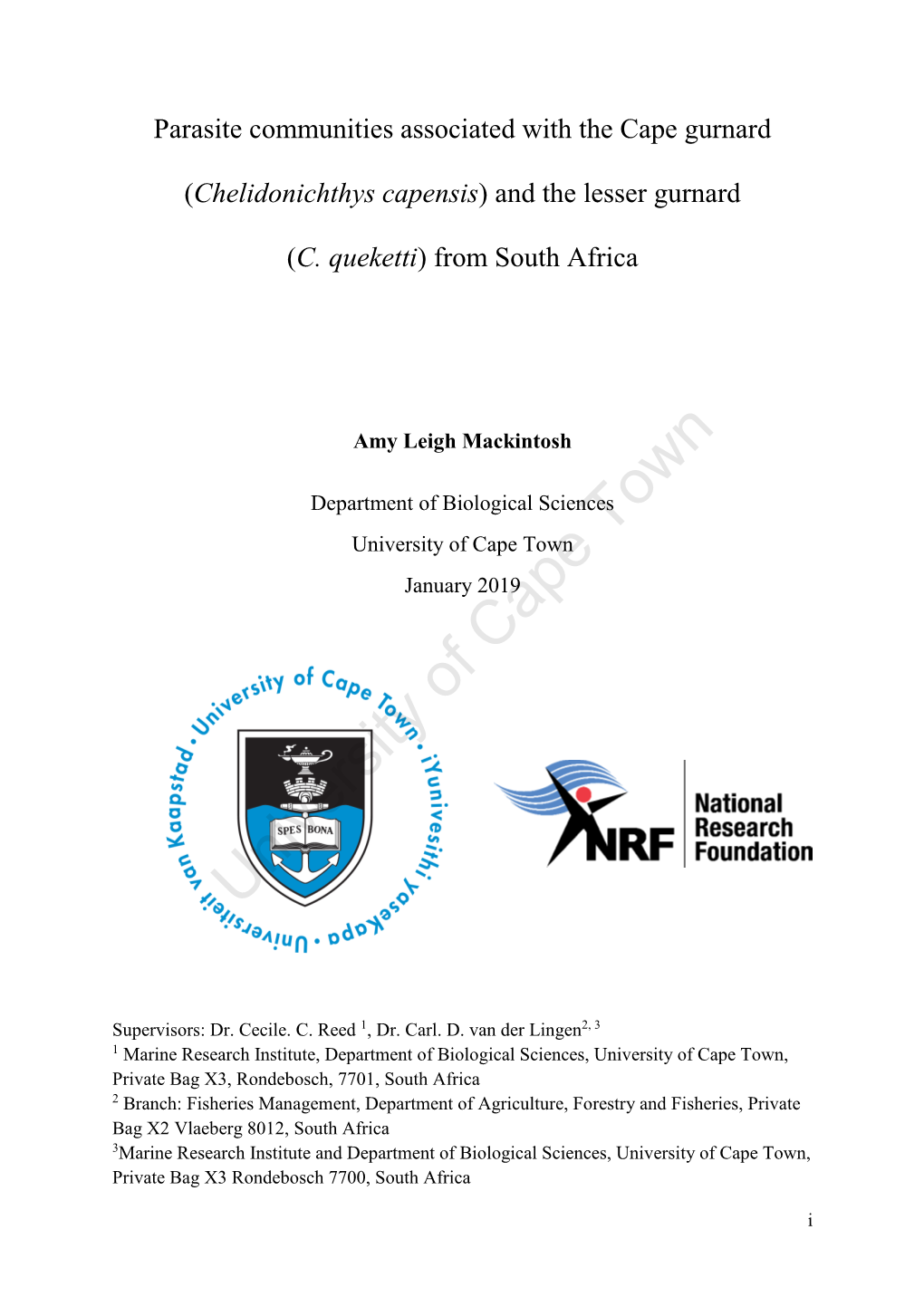
Load more
Recommended publications
-
An Eclectic Overview of the SA Marine Science Community
A biologists’ personal overview of the SA Marine Science community and its outputs (2001-2006)*1 Mark J Gibbons, Department of Biodiversity and Conservation Biology, University of the Western Cape, Private Bag X17, Bellville 7535, South Africa. Email: [email protected] ABSTRACT I have analysed 1 295 of the outputs published by the South African Marine Science Community (SAMSC) in either peer-reviewed journals or as books/book chapters for the period 2001-2006, with a view to identifying trends in the field, summarizing institutional overlaps and assessing the demographic state-of-play. Although almost 70% of our outputs were published internationally, we published the bulk of our research in strictly marine science journals which suggests, perhaps, that we need to be thinking bigger. More than 22% of all outputs were led by International colleagues, and these were published in journals with a significantly higher Impact Factor than those led by local authors. Women led less than 25%, and persons from previously disadvantaged backgrounds led less than 10%, of all outputs: this needs monitoring, discussion and pro-active response by employers. Thirty-six authors (~95% male, ~97% white) were responsible for more than 50% of all outputs, which suggests that the field is not totally dominated by an ageing cohort. Despite the fact that the bulk of the SAMSC is centred in the SW Cape, our study areas are approximately equally spread around the coastline, though there is an obvious institutional bias to the geographical location of study sites. Globally-orientated studies were generally published in “better” outlets than locally-orientated work, and were more likely to be led by international colleagues. -
Crustacea, Isopoda) from the Indian Ocean Coast of South Africa, with a Key to the Externally Attaching Genera of Cymothoidae
A peer-reviewed open-access journal ZooKeys 889: 1–15 (2019) Bambalocra, a new genus of Cymothoidae 1 doi: 10.3897/zookeys.889.38638 RESEARCH ARTICLE http://zookeys.pensoft.net Launched to accelerate biodiversity research A new genus and species of fish parasitic cymothoid (Crustacea, Isopoda) from the Indian Ocean coast of South Africa, with a key to the externally attaching genera of Cymothoidae Niel L. Bruce1,2, Rachel L. Welicky2,3, Kerry A. Hadfield2, Nico J. Smit2 1 Biodiversity & Geosciences Program, Queensland Museum, PO Box: 3300, South Brisbane BC, Queensland 4101, Australia 2 Water Research Group, Unit for Environmental Sciences and Management, North-West University, Private Bag X6001, Potchefstroom, 2520, South Africa 3 School of Aquatic and Fishery Sciences, University of Washington, 1122 NE Boat Street, Seattle, WA, 98105, USA Corresponding author: Niel L. Bruce ([email protected]) Academic editor: Saskia Brix | Received 30 July 2019 | Accepted 9 October 2019 | Published 14 November 2019 http://zoobank.org/88E937E5-7C48-49F8-8260-09872CB08683 Citation: Bruce NL, Welicky RL, Hadfield KA, Smit NJ (2019) A new genus and species of fish parasitic cymothoid (Crustacea, Isopoda) from the Indian Ocean coast of South Africa, with a key to the externally attaching genera of Cymothoidae. ZooKeys 889: 1–15. https://doi.org/10.3897/zookeys.889.38638 Abstract Bambalocra intwala gen. et sp. nov. is described from Sodwana Bay, north-eastern South Africa. The monotypic genus is characterised by the broadly truncate anterior margin of the head with a ventral ros- trum, coxae 2–5 being ventral in position not forming part of the body outline and not or barely visible in dorsal view, and the posterolateral margins of pereonites 6 and 7 are posteriorly produced and broadly rounded. -
Trigloporus Lastoviza, Bonnaterre, 1788
International Journal of Fisheries and Aquatic Studies 2015; 3(1): 75-80 ISSN: 2347-5129 (ICV-Poland) Impact Value: 5.62 Age, growth and mortality of Streaked Gurnard (GIF) Impact Factor: 0.352 IJFAS 2015; 3(1): 75-80 © 2015 IJFAS (Trigloporus lastoviza, Bonnaterre, 1788) in the Egyptian www.fisheriesjournal.com Mediterranean waters off Alexandria Received: 11-06-2015 Accepted: 13-07-2015 Sabry S El-Serafy, Fahmy I El-Gammal, Sahar F Mehanna, Nasr-Allah H Sabry S El-Serafy Zoology Department, Faculty of Abdel- Hameid, Elsayed FE Farrag Science, Benha University. Abstract Fahmy I El-Gammal Age, growth and mortality of Streaked Gurnard Trigloporus lastoviza from the Egyptian Mediterranean Fisheries Department, National waters off Alexandria were investigated between the period from July 2009 and August 2010. The Institute of Oceanography and observed maximum age was 4 years for both sexes based on otolith readings. The length-weight Fisheries. relationship was estimated as W=0.0088L3.0694 (r=0.9854); W=0.0085L3.0836 (r=0.9803) and W=0.0106L3.0058 (r=0.9853) for males, females and combined sexes respectively. The von Bertalanffy Sahar F Mehanna Fisheries Department, National growth equations for length were: Lt=27.17 (1-e-0.3466 (t+1.01), Lt = 27.0 (1 - e -0.3703 (t + 0.93) and Institute of Oceanography and Lt = 26.92 (1 - e -0.3699 (t + 0.92) for males, females and combined sexes respectively. The growth Fisheries. performance index value (Φ) was calculated as 2.41, 2.43 and 2.43 for males, females and combined sexes respectively. -
Investigating the Biological and Socio-Economic Impacts of Marine
Investigating the biological and socio-economic impacts of marine protected area network design in Europe Submitted by Kristian Metcalfe January 2013 to the Durrell Institute of Conservation and Ecology, School of Anthropology and Conservation, University of Kent as a thesis for the degree of Doctor of Philosophy in Biodiversity Management SUPERVISORS Dr Robert J Smith - Durrell Institute of Conservation and Ecology (DICE) Dr Sandrine Vaz - Institut Français de Recherche pour l‟exploitation de la Mer (IFREMER) Professor Stuart R Harrop - Durrell Institute of Conservation and Ecology (DICE) ii ABSTRACT Marine ecosystems are under increasing pressure from a diverse range of threats. Many national governments have responded to these threats by establishing marine protected area (MPA) networks. One such approach for designing MPA networks is systematic conservation planning, which is now considered the most effective system for designing protected area networks. However, the main exception to this trend is Europe, where the designation of MPAs is still largely based on expert opinion, despite growing awareness that these existing methods are not the most effective. Therefore, there is a need to demonstrate how systematic conservation planning can be used to inform MPA design in European waters and show how this approach can fit within existing marine conservation policy and practice. This thesis brings together a range of biological, legal and socio-economic data to address these issues and is comprised of four main chapters: After the introductory chapter, this thesis begins with a review of how existing approaches for guiding the selection of MPAs in Europe compare to conservation planning best practice (Chapter 2). -
TNP SOK 2011 Internet
GARDEN ROUTE NATIONAL PARK : THE TSITSIKAMMA SANP ARKS SECTION STATE OF KNOWLEDGE Contributors: N. Hanekom 1, R.M. Randall 1, D. Bower, A. Riley 2 and N. Kruger 1 1 SANParks Scientific Services, Garden Route (Rondevlei Office), PO Box 176, Sedgefield, 6573 2 Knysna National Lakes Area, P.O. Box 314, Knysna, 6570 Most recent update: 10 May 2012 Disclaimer This report has been produced by SANParks to summarise information available on a specific conservation area. Production of the report, in either hard copy or electronic format, does not signify that: the referenced information necessarily reflect the views and policies of SANParks; the referenced information is either correct or accurate; SANParks retains copies of the referenced documents; SANParks will provide second parties with copies of the referenced documents. This standpoint has the premise that (i) reproduction of copywrited material is illegal, (ii) copying of unpublished reports and data produced by an external scientist without the author’s permission is unethical, and (iii) dissemination of unreviewed data or draft documentation is potentially misleading and hence illogical. This report should be cited as: Hanekom N., Randall R.M., Bower, D., Riley, A. & Kruger, N. 2012. Garden Route National Park: The Tsitsikamma Section – State of Knowledge. South African National Parks. TABLE OF CONTENTS 1. INTRODUCTION ...............................................................................................................2 2. ACCOUNT OF AREA........................................................................................................2 -
Triple Barcoding for a Hyperparasite, Its Parasitic Host, and the Host Itself
Parasite 28, 49 (2021) Ó C. Bouguerche et al., published by EDP Sciences, 2021 https://doi.org/10.1051/parasite/2021044 Available online at: www.parasite-journal.org RESEARCH ARTICLE OPEN ACCESS Triple barcoding for a hyperparasite, its parasitic host, and the host itself: a study of Cyclocotyla bellones (Monogenea) on Ceratothoa parallela (Isopoda) on Boops boops (Teleostei) Chahinez Bouguerche1,4, Fadila Tazerouti1, Delphine Gey2,3, and Jean-Lou Justine4,* 1 Université des Sciences et de la Technologie Houari Boumediene, Faculté des Sciences Biologiques, Laboratoire de Biodiversité et Environnement : Interactions – Génomes, BP 32, El Alia, Bab Ezzouar, Algiers, Algeria 2 Service de Systématique moléculaire, UMS 2700 CNRS, Muséum National d’Histoire Naturelle, Sorbonne Universités, 43 rue Cuvier, CP 26, 75231 Paris Cedex 05, France 3 UMR7245 MCAM, Muséum National d’Histoire Naturelle, 43 rue Cuvier, CP 52, 75231 Paris Cedex 05, France 4 Institut Systématique Évolution Biodiversité (ISYEB), Muséum National d’Histoire Naturelle, CNRS, Sorbonne Université, EPHE, Université des Antilles, 43 rue Cuvier, CP 51, 75231 Paris Cedex 05, France Received 15 October 2020, Accepted 7 May 2021, Published online 7 June 2021 Abstract – Cyclocotyla bellones Otto, 1823 (Diclidophoridae) is a monogenean characterised by an exceptional way of life. It is a hyperparasite that attaches itself to the dorsal face of isopods, themselves parasites in the buccal cavity of fishes. In this study, Cy. bellones was found on Ceratothoa parallela (Otto, 1828), a cymothoid isopod parasite of the sparid fish Boops boops off Algeria in the Mediterranean Sea. We provide, for the first time, molecular barcoding information of a hyperparasitic monogenean, the parasitic crustacean host, and the fish host, with COI sequences. -
A Revision of the Chameleon Species Chamaeleo Pfeili Schleich
A revision of the chameleon species Chamaeleo pfeili Schleich (Squamata; Chamaeleonidae) with description of a new material of chamaeleonids from the Miocene deposits of southern Germany ANDREJ ÈERÒANSKÝ A revision of Chamaeleo pfeili Schleich is presented. The comparisons of the holotypic incomplete right maxilla with those of new specimens described here from the locality Langenau (MN 4b) and of the Recent species of Chamaeleo, Furcifer and Calumma is carried out. It is shown that the type material of C. pfeili and the material described here lack autapomorphic features. Schleich based his new species on the weak radial striations on the apical parts of bigger teeth. However, this character is seen in many species of extant chameleons, e.g. Calumma globifer, Furcifer pardalis and C. chamaeleon. For this reason, the name C. pfeili is considered a nomen dubium. This paper provides detailed descrip- tions and taxonomy of unpublished material from Petersbuch 2 (MN 4a) and Wannenwaldtobel (MN 5/6) in Germany. The material is only fragmentary and includes jaw bits. The morphology of the Petersbuch 2 material is very similar to that of the chameleons described from the Czech Republic. • Key words: Chamaeleo pfeili, nomen dubium, morphology, Wannenwaldtobel, Petersbuch 2, Langenau, Neogene. ČERŇANSKÝ, A. 2011. A revision of the chameleon species Chamaeleo pfeili Schleich (Squamata; Chamaeleonidae) with description of a new material of chamaeleonids from the Miocene deposits of southern Germany. Bulletin of Geosciences 86(2), 275–282 (6 figures). Czech Geological Survey, Prague. ISSN 1214-1119. Manuscript received Feb- ruary 11, 2011; accepted in revised form March 21, 2011; published online April 20, 2011; issued June 20, 2011. -
Miscellaneous Demersal Fishes Capture Production by Species, Fishing Areas and Countries Or Areas B-34 Poissons Démersaux Diver
173 Miscellaneous demersal fishes Capture production by species, fishing areas and countries or areas B-34 Poissons démersaux divers Captures par espèces, zones de pêche et pays ou zones Peces demersales diversos Capturas por especies, áreas de pesca y países o áreas Species, Fishing area Espèce, Zone de pêche 1997 1998 1999 2000 2001 2002 2003 2004 2005 2006 Especie, Área de pesca t t t t t t t t t t Greater argentine ...B ...C Argentina silus 1,23(05)015,03 ARU 27 Netherlands ... ... ... ... ... ... 2 611 10 662 3 637 1 062 Norway ... ... ... ... ... ... ... ... ... 1 820 27 Fishing area total ... ... ... ... ... ... 2 611 10 662 3 637 2 882 Species total ... ... ... ... ... ... 2 611 10 662 3 637 2 882 Argentine ...B ...C Argentina sphyraena 1,23(05)015,04 ARY 27 Netherlands ... ... ... ... ... ... - - 2 232 3 566 Norway ... ... ... ... ... ... ... ... ... 2 584 27 Fishing area total ... ... ... ... ... ... ... ... 2 232 6 150 Species total ... ... ... ... ... ... ... ... 2 232 6 150 Argentines Argentines Argentinas Argentina spp 1,23(05)015,XX ARG 21 Canada 591 51 12 8 17 20 12 1 3 1 Cuba 553 4 5 - - - - - - - Russian Fed - - - 5 - - - - - - 21 Fishing area total 1 144 55 17 13 17 20 12 1 3 1 27 Denmark 1 455 748 1 420 1 039 916 614 918 910 470 335 Faroe Is 8 433 17 167 8 186 6 388 9 572 7 058 6 264 3 441 7 055 12 576 France - - 114 55 41 1 - 147 11 - Germany 1 498 633 24 483 189 150 164 1 086 181 219 Iceland 3 367 13 387 5 495 4 595 2 478 4 357 2 680 3 645 - 4 776 Ireland 1 089 405 396 4 709 7 505 7 592 96 82 20 - Netherlands 4 696 4 964 8 033 3 636 3 659 4 213 - - - - Norway 5 167 8 654 7 823 6 107 14 876 7 406 8 351 11 577 17 073 20 744 Portugal - - - - - - - - - 0 Russian Fed - - - 1 214 496 293 154 721 79 39 Spain - - - 34 34 3 7 18 19 50 Sweden 541 428 0 273 1 010 484 42 0 - 0 UK - - 28 - 7 955 4 862 109 579 75 5 27 Fishing area total 26 246 46 386 31 519 28 533 48 731 37 033 18 785 22 206 24 983 38 744 34 Morocco - - - - - - - 231 295 509 34 Fishing area total - - - - - - - 231 295 509 37 France - - 7 4 4 7 5 7 6 5 Morocco .. -
Trawl Survey of Red Gurnard, John Dory, Tarakihi and Associated Species Off the Bay of Plenty, North Island, February 1999 (KAH9902)
4 IWA Taihoro Nukurangi Trawl survey of red gurnard, John dory, tarakihi and associated species off the Bay of Plenty, North Island, February 1999 (KAH9902) M. Morrison, D. Parkinson Final Research Report for Ministry of Fisheries Research Project INT9803 National Institute of Water and Atmospheric Research November 1999 Final Research Report Report Title: Trawl survey of red gurnard, John dory, terakihi and associated species off the Bay of Plenty, North Island, February 1999 (KAH9902) Authors: M. Morrison, D. Parkinson 1. Date: 15/10/99 2. Contractor: NIWA 3. Project Title: Estimation of inshore fish abundance in the Bay of Plenty using trawl surveys 4. Project Code: INT9803 5. Project Leader: M. Morrison 6. Duration of Project: Start Date 1 October 1998 Expected End Date 30 September 1999 7. Executive Summary: A trawl survey of the Bay of Plenty was successfully completed during February 1999. Seventy eight stations were completed (50 phase 1, 28 phase 2) within 8 depth and area strata. Target c.v.s on biomass estimates were 15% for GUR, 20% for JDO, and 30% for TAR; achieved c.v.s were 14%, 14%, and 27% respectively. Length and weight information was also collected for all other QMS species in the catch, and for leatherjacket, frostfish, kahawai and kingfish. Red gurnard were caught throughout the Bay of Plenty, with relatively large individual catches occurring in the 10-100 m depth range. John dory were less common, with scattered larger catches. Relatively few tarakihi were encountered; most of the tows catching these fish were in deeper water in the middle of the survey area. -
Marine Fishes from Galicia (NW Spain): an Updated Checklist
1 2 Marine fishes from Galicia (NW Spain): an updated checklist 3 4 5 RAFAEL BAÑON1, DAVID VILLEGAS-RÍOS2, ALBERTO SERRANO3, 6 GONZALO MUCIENTES2,4 & JUAN CARLOS ARRONTE3 7 8 9 10 1 Servizo de Planificación, Dirección Xeral de Recursos Mariños, Consellería de Pesca 11 e Asuntos Marítimos, Rúa do Valiño 63-65, 15703 Santiago de Compostela, Spain. E- 12 mail: [email protected] 13 2 CSIC. Instituto de Investigaciones Marinas. Eduardo Cabello 6, 36208 Vigo 14 (Pontevedra), Spain. E-mail: [email protected] (D. V-R); [email protected] 15 (G.M.). 16 3 Instituto Español de Oceanografía, C.O. de Santander, Santander, Spain. E-mail: 17 [email protected] (A.S); [email protected] (J.-C. A). 18 4Centro Tecnológico del Mar, CETMAR. Eduardo Cabello s.n., 36208. Vigo 19 (Pontevedra), Spain. 20 21 Abstract 22 23 An annotated checklist of the marine fishes from Galician waters is presented. The list 24 is based on historical literature records and new revisions. The ichthyofauna list is 25 composed by 397 species very diversified in 2 superclass, 3 class, 35 orders, 139 1 1 families and 288 genus. The order Perciformes is the most diverse one with 37 families, 2 91 genus and 135 species. Gobiidae (19 species) and Sparidae (19 species) are the 3 richest families. Biogeographically, the Lusitanian group includes 203 species (51.1%), 4 followed by 149 species of the Atlantic (37.5%), then 28 of the Boreal (7.1%), and 17 5 of the African (4.3%) groups. We have recognized 41 new records, and 3 other records 6 have been identified as doubtful. -
Companion Reptile Care SERIES the Veiled Chameleon
cham.qxd 8/10/2007 3:43 PM Page 1 VEILED Most Common Disorders The veiled chameleon of Veiled Chameleons (Chamaeleo calyptratus) is a •Dystocia (egg-binding) large, colorful, and robust lizard •Metabolic bone disease indigenous to coastal regions of CHAMELEON •Toenail loss / foot infections Yemen and Saudi Arabia. Now well •Intestinal parasites established in captivity, it is one •Respiratory / sinus / ocular infections of the most popular and widely •Stomatitis / periodontal disease recommended chameleons for •Abscesses / cellulitis / osteomyelitis the novice reptile keeper. •Loss of tongue function •Kidney disease A characteristic feature of this •Hemipene prolapse species is the impressively high •Dehydration casque on the head. Adult males have a higher casque than females. Having your veiled chameleon examined on a regular basis Some authorities have suggested that by an exotic animal veterinarian who is familiar with the casque may serve to collect reptiles can prevent many of the common disorders above. and channel water, such as morning dewdrops or fog, into the mouth. Others believe that it functions to dissipate heat. A more recent hypothesis suggests that it may amplify a low frequency “buzzing” used by this species to communicate with one another. Veiled chameleons also possess prehensile tails, long whip-like tongues, independently moving eyes, zygodactyl feet, and a spectacular array of changing colors. Zoological Education Network provides educational ©2007 Zoological Education Network materials about exotic companion animals. 800-946-4782 561-641-6745 www.exoticdvm.com CompanionC Reptile Care SERIES cham.qxd 8/10/2007 3:43 PM Page 2 What Your Veterinarian Looks for in a Healthy Veiled Chameleon Active and alert What to Expect from Your Veiled Chameleon Eyes open and clear attitude hameleons are unique, attractive and fascinating 24 hours before feeding them out. -
No Longer Single! Description of Female Calumma Vatosoa (Squamata, Chamaeleonidae) Including a Review of the Species and Its Systematic Position
Zoosyst. Evol. 92 (1) 2016, 13–21 | DOI 10.3897/zse.92.6464 museum für naturkunde No longer single! Description of female Calumma vatosoa (Squamata, Chamaeleonidae) including a review of the species and its systematic position David Prötzel1, Bernhard Ruthensteiner1, Frank Glaw1 1 Zoologische Staatssammlung München (ZSM-SNSB), Münchhausenstr. 21, 81247 München, Germany http://zoobank.org/CFD64DFB-D085-4D1A-9AA9-1916DB6B4043 Corresponding author: David Prötzel ([email protected]) Abstract Received 3 September 2015 Calumma vatosoa is a Malagasy chameleon species that has until now been known only Accepted 26 November 2015 from the male holotype and a photograph of an additional male specimen. In this paper Published 8 January 2016 we describe females of the chameleon Calumma vatosoa for the first time, as well as the skull osteology of this species. The analysed females were collected many years before Academic editor: the description of C. vatosoa, and were originally described as female C. linotum. Ac- Johannes Penner cording to external morphology, osteology, and distribution these specimens are assigned to C. vatosoa. Furthermore we discuss the species group assignment of C. vatosoa and transfer it from the C. furcifer group to the C. nasutum group. A comparison of the exter- Key Words nal morphology of species of both groups revealed that C. vatosoa has a relatively shorter distance from the anterior margin of the orbit to the snout tip, more heterogeneous scala- Madagascar tion at the lower arm, a significantly lower number of supralabial and infralabial scales, chameleon and a relatively longer tail than the members of the C. furcifer group.