Generation of Motor Neurons from Pluripotent Stem Cells
Total Page:16
File Type:pdf, Size:1020Kb
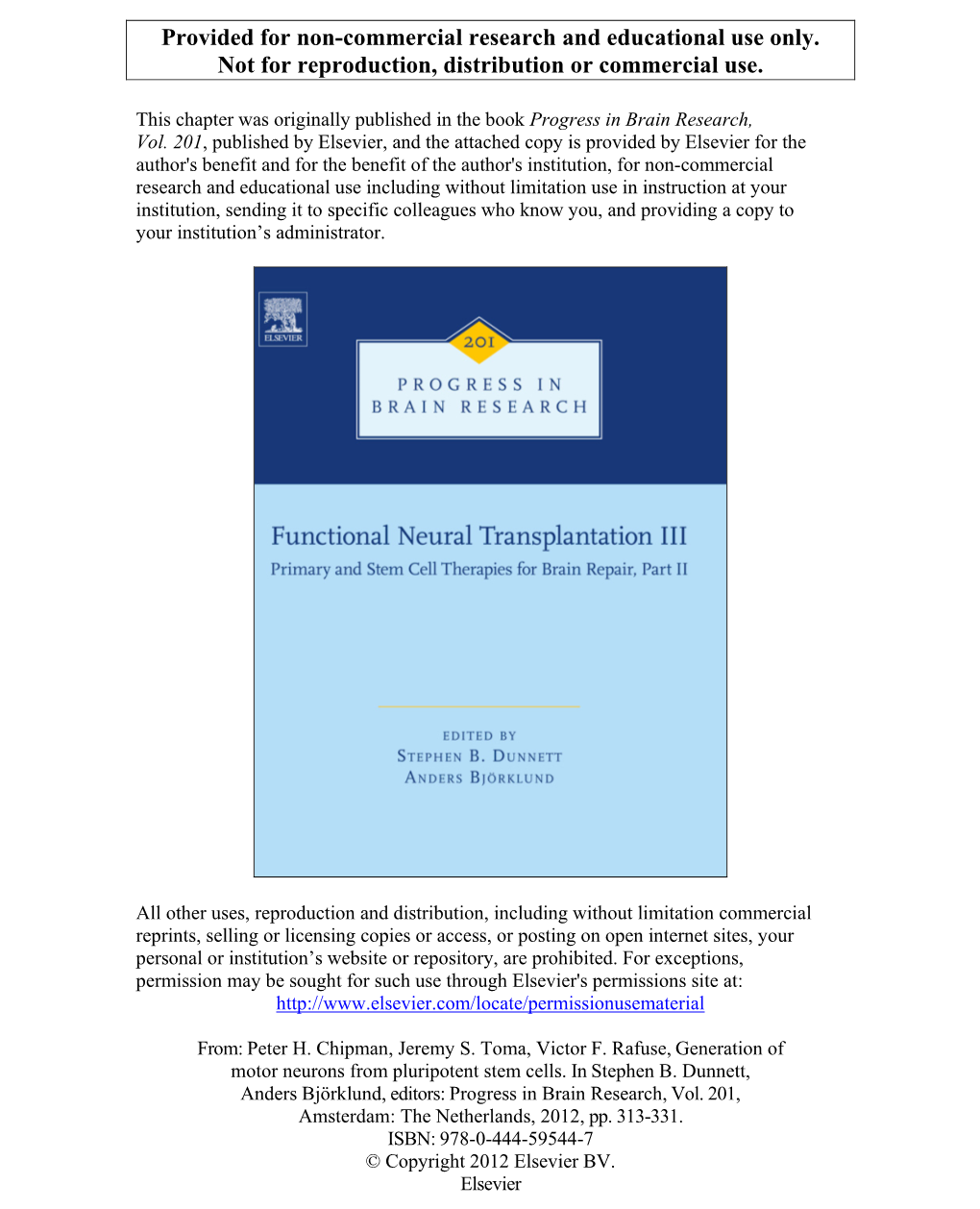
Load more
Recommended publications
-
Induction of Motor Neurons by Sonic Hedgehog Is Independent of Floor Plate Differentiation Yasuto Tanabe, Henk Roelink and Thomas M
View metadata, citation and similar papers at core.ac.uk brought to you by CORE provided by Elsevier - Publisher Connector Induction of motor neurons by Sonic hedgehog is independent of floor plate differentiation Yasuto Tanabe, Henk Roelink and Thomas M. Jessell Howard Hughes Medical Institute, Deptartment of Biochemistry and Molecular Biophysics, Center for Neurobiology and Behavior, Columbia University, 701 West 168th Street, New York, New York 10032, USA. Background: The differentiation of floor plate cells and transfected with Shh induced both floor plate cells and motor neurons in the vertebrate neural tube appears to be motor neurons when grown in contact with neural plate induced by signals from the notochord. The secreted pro- explants, whereas only motor neurons were induced when tein encoded by the Sonic hedgehog (Shh) gene is expressed the explants were grown at a distance from Shh-trans- by axial midline cells and can induce floor plate cells in fected COS cells. Direct transfection of neural plate cells vivo and in vitro. Motor neurons can also be induced in with an Shh-expression construct induced both floor plate vitro by cells that synthesize Sonic hedgehog protein cells and motor neurons, with motor neuron differentia- (Shh). It remains unclear, however, if the motor-neuron- tion occurring prior to, or coincidentally with, floor plate inducing activity of Shh depends on the synthesis of a dis- differentiation. The induction of motor neurons appears, tinct signaling molecule by floor plate cells. To resolve therefore, not to depend on floor plate differentiation. this issue, we have developed an in vitro assay which Conclusions: The induction of motor neurons by uncouples the notochord-mediated induction of motor Shh does not depend on distinct floor-plate-derived neurons from floor plate differentiation, and have used signaling molecules. -
Oligodendrocytes in Development, Myelin Generation and Beyond
cells Review Oligodendrocytes in Development, Myelin Generation and Beyond Sarah Kuhn y, Laura Gritti y, Daniel Crooks and Yvonne Dombrowski * Wellcome-Wolfson Institute for Experimental Medicine, Queen’s University Belfast, Belfast BT9 7BL, UK; [email protected] (S.K.); [email protected] (L.G.); [email protected] (D.C.) * Correspondence: [email protected]; Tel.: +0044-28-9097-6127 These authors contributed equally. y Received: 15 October 2019; Accepted: 7 November 2019; Published: 12 November 2019 Abstract: Oligodendrocytes are the myelinating cells of the central nervous system (CNS) that are generated from oligodendrocyte progenitor cells (OPC). OPC are distributed throughout the CNS and represent a pool of migratory and proliferative adult progenitor cells that can differentiate into oligodendrocytes. The central function of oligodendrocytes is to generate myelin, which is an extended membrane from the cell that wraps tightly around axons. Due to this energy consuming process and the associated high metabolic turnover oligodendrocytes are vulnerable to cytotoxic and excitotoxic factors. Oligodendrocyte pathology is therefore evident in a range of disorders including multiple sclerosis, schizophrenia and Alzheimer’s disease. Deceased oligodendrocytes can be replenished from the adult OPC pool and lost myelin can be regenerated during remyelination, which can prevent axonal degeneration and can restore function. Cell population studies have recently identified novel immunomodulatory functions of oligodendrocytes, the implications of which, e.g., for diseases with primary oligodendrocyte pathology, are not yet clear. Here, we review the journey of oligodendrocytes from the embryonic stage to their role in homeostasis and their fate in disease. We will also discuss the most common models used to study oligodendrocytes and describe newly discovered functions of oligodendrocytes. -
Sonic Hedgehog Induces the Lateral Floor Plate
Development 129, 4785-4796 (2002) 4785 Printed in Great Britain © The Company of Biologists Limited 2002 DEV2912 Dual origin of the floor plate in the avian embryo Jean-Baptiste Charrier, Françoise Lapointe, Nicole M. Le Douarin and Marie-Aimée Teillet* Institut d’Embryologie Cellulaire et Moléculaire, CNRS and Collège de France, UMR 7128, 49bis Avenue de la Belle Gabrielle, 94736 Nogent-sur-Marne Cedex, France *Author for correspondence (e-mail: [email protected]) Accepted 30 July 2002 SUMMARY Molecular analysis carried out on quail-chick chimeras, in development, one can experimentally obtain a complete which quail Hensen’s node was substituted for its chick floor plate in the neural epithelium by the inductive action counterpart at the five- to six-somite stage (ss), showed that of either a notochord or a MFP. The competence of the the floor plate of the avian neural tube is composed of neuroepithelium to respond to notochord or MFP signals is distinct areas: (1) a median one (medial floor plate or MFP) restricted to a short time window, as only the posterior-most derived from Hensen’s node and characterised by the same region of the neural plate of embryos younger than 15 ss is gene expression pattern as the node cells (i.e. expression of able to differentiate a complete floor plate comprising MFP HNF3β and Shh to the exclusion of genes early expressed and LFP. Moreover, MFP differentiation requires between in the neural ectoderm such as CSox1); and (2) lateral 4 and 5 days of exposure to the inducing tissues. -
Induction of Floor Plate Differentiation by Contact-Dependent
Development 117, 205-218 (1993) 205 Printed in Great Britain © The Company of Biologists Limited 1993 Induction of floor plate differentiation by contact-dependent, homeogenetic signals Marysia Placzek1, Thomas M. Jessell2 and Jane Dodd1,* 1Department of Physiology and Cellular Biophysics, 2Department of Biochemistry and Molecular Biophysics and Howard Hughes Medical Institute, and 1,2Center for Neurobiology and Behavior, Columbia University, New York, New York 10032, USA *Author for correspondence SUMMARY The floor plate is located at the ventral midline of the derive from medial floor plate cells. The response of neural tube and has been implicated in neural cell pat- neural plate cells to inductive signals declines with terning and axon guidance. To address the cellular embryonic age, suggesting that the mediolateral extent mechanisms involved in floor plate differentiation, we of the floor plate is limited by a loss of competence of have used an assay that monitors the expression of floor- neural cells. The rostral boundary of the floor plate at plate-specific antigens in neural plate explants cultured the midbrain-forebrain junction appears to result from in the presence of inducing tissues. Contact-mediated the lack of inducing activity in prechordal mesoderm signals from both the notochord and the floor plate act and the inability of rostral neural plate cells to respond directly on neural plate cells to induce floor plate differ- to inductive signals. entiation. Floor plate induction is initiated medially by a signal from the notochord, but appears to be propa- gated to more lateral cells by homeogenetic signals that Key words: floor plate, notochord, neural plate, induction. -
Ectoderm: Neurulation, Neural Tube, Neural Crest
4. ECTODERM: NEURULATION, NEURAL TUBE, NEURAL CREST Dr. Taube P. Rothman P&S 12-520 [email protected] 212-305-7930 Recommended Reading: Larsen Human Embryology, 3rd Edition, pp. 85-102, 126-130 Summary: In this lecture, we will first consider the induction of the neural plate and the formation of the neural tube, the rudiment of the central nervous system (CNS). The anterior portion of the neural tube gives rise to the brain, the more caudal portion gives rise to the spinal cord. We will see how the requisite numbers of neural progenitors are generated in the CNS and when these cells become post mitotic. The molecular signals required for their survival and further development will also be discussed. We will then turn our attention to the neural crest, a transient structure that develops at the site where the neural tube and future epidermis meet. After delaminating from the neuraxis, the crest cells migrate via specific pathways to distant targets in an embryo where they express appropriate target-related phenotypes. The progressive restriction of the developmental potential of crest-derived cells will then be considered. Additional topics include formation of the fundamental subdivisions of the CNS and PNS, as well as molecular factors that regulate neural induction and regional distinctions in the nervous system. Learning Objectives: At the conclusion of the lecture you should be able to: 1. Discuss the tissue, cellular, and molecular basis for neural induction and neural tube formation. Be able to provide some examples of neural tube defects caused by perturbation of neural tube closure. -
A Revised Model of Xenopus Dorsal Midline Development: Differential and Separable Requirements for Notch and Shh Signaling
View metadata, citation and similar papers at core.ac.uk brought to you by CORE provided by Elsevier - Publisher Connector Developmental Biology 352 (2011) 254–266 Contents lists available at ScienceDirect Developmental Biology journal homepage: www.elsevier.com/developmentalbiology A revised model of Xenopus dorsal midline development: Differential and separable requirements for Notch and Shh signaling Sara M. Peyrot a,b,1, John B. Wallingford a,b,c, Richard M. Harland a,⁎ a Dept. of Molecular and Cell Biology and Center for Integrative Genomics, University of California, Berkeley, CA 94720, USA b Dept. of Molecular Cell and Developmental Biology, University of Texas, Austin, TX 78712, USA c Howard Hughes Medical Institute, USA article info abstract Article history: The development of the vertebrate dorsal midline (floor plate, notochord, and hypochord) has been an area of Received for publication 8 November 2010 classical research and debate. Previous studies in vertebrates have led to contrasting models for the roles of Revised 18 January 2011 Shh and Notch signaling in specification of the floor plate, by late inductive or early allocation mechanisms, Accepted 19 January 2011 respectively. Here, we show that Notch signaling plays an integral role in cell fate decisions in the dorsal Available online 27 January 2011 midline of Xenopus laevis, similar to that observed in zebrafish and chick. Notch signaling promotes floor plate and hypochord fates over notochord, but has variable effects on Shh expression in the midline. In contrast to Keywords: fi fl Floor plate previous reports in frog, we nd that Shh signaling is not required for oor plate vs. -
Induction of the Oligodendrocyte Lineage by Ventral Midline Cells and Sonic Hedgehog
DEVELOPMENTAL BIOLOGY 177, 30±42 (1996) ARTICLE NO. 0142 Determination of Neuroepithelial Cell Fate: Induction of the Oligodendrocyte Lineage by Ventral Midline Cells and Sonic Hedgehog Nigel P. Pringle,1 Wei-Ping Yu,1,2 Sarah Guthrie,* Henk Roelink,² Andrew Lumsden,* Alan C. Peterson,³ and William D. Richardson3 MRC Laboratory for Molecular Cell Biology and Department of Biology, University College London, Gower Street, London WC1E 6BT, United Kingdom; *Division of Anatomy and Cell Biology, United Medical and Dental Schools, Guy's Hospital Campus, St. Thomas Street, London Bridge, London SE1 9RT, United Kingdom; ³Molecular Oncology Group, Royal Victoria Hospital, McGill University, Hershey Pavilion (H5), 687 Pine Avenue West, Montreal, Quebec H3A 1A1, Canada; and ²Department of Biological Structures, University of Washington School of Medicine, P.O. Box 537420 Seattle, Washington 98195 Near the ¯oor plate of the embryonic neural tube there is a group of neuroepithelial precursor cells that are specialized for production of the oligodendrocyte lineage. We performed experiments to test whether speci®cation of these neuroepithelial oligodendrocyte precursors, like other ventral neural cell types, depends on signals from the notochord and/or ¯oor plate. We analyzed heterozygous Danforth's short tail (Sd//) mutant mice, which lack a notochord and ¯oor plate in caudal regions of the neural tube, and found that oligodendrocyte precursors did not appear at the ventricular surface where there was no ¯oor plate. Moreover, oligodendrocytes did not develop in explant cultures of Sd// spinal cord in the absence of a ¯oor plate. When a second notochord was grafted into an ectopic position dorsolateral to the endogenous notochord of a chicken embryo, an additional ¯oor plate was induced along with an ectopic focus of oligodendrocyte precursors at the ventricular surface. -
Distinct Modes of Floor Plate Induction in the Chick Embryo
View metadata, citation and similar papers at core.ac.uk brought to you by CORE provided by Caltech Authors Research article 4809 Distinct modes of floor plate induction in the chick embryo Iain Patten1,*, Paul Kulesa2,†, Michael M. Shen3, Scott Fraser2 and Marysia Placzek1,‡ 1Centre for Developmental Genetics, Department of Biomedical Science, University of Sheffield, Sheffield S10 2TN, UK 2Beckman Institute 139-74, California Institute of Technology, Pasadena, CA 91125, USA 3Center for Advanced Biotechnology and Medicine, UMDNJ-Robert Wood Johnson Medical School, 679 Hoes Lane, Piscataway, NJ 08854, USA *Present address: Departament de Genetica, Facultat de Biologia, Universitat de Barcelona, Avenida Diagonal 645, Barcelona 08028, Spain †Present address: Stowers Institute for Medical Research, 1000 East 50th Street, Kansas City, MO 64110, USA ‡Author for correspondence (e-mail: [email protected]) Accepted 19 June 2003 Development 130, 4809-4821 © 2003 The Company of Biologists Ltd doi:10.1242/dev.00694 Summary To begin to reconcile models of floor plate formation in the nodal homologue, is expressed in the nascent prechordal vertebrate neural tube, we have performed experiments mesoderm and we provide evidence that Nodal signalling aimed at understanding the development of the early floor can cooperate with Shh to induce the epiblast precursors plate in the chick embryo. Using real-time analyses of cell to a floor-plate fate. These results indicate that a shared behaviour, we provide evidence that the principal lineage with axial mesoderm cells is not a pre-requisite for contributor to the early neural midline, the future anterior floor plate differentiation and suggest parallels between the floor plate, exists as a separate population of floor plate development of the floor plate in amniote and anamniote precursor cells in the epiblast of the gastrula stage embryo, embryos. -
Distinct Regulators of Shh Transcription in the Floor Plate and Notochord
Development 130, 3891-3902 3891 © 2003 The Company of Biologists Ltd doi:10.1242/dev.00590 Distinct regulators of Shh transcription in the floor plate and notochord indicate separate origins for these tissues in the mouse node Yongsu Jeong and Douglas J. Epstein Department of Genetics, University of Pennsylvania School of Medicine, Clinical Research Building, Room 470, 415 Curie Blvd, Philadelphia, PA 19104, USA *Author for correspondence (e-mail: [email protected]) Accepted 12 May 2003 SUMMARY The establishment of the floor plate at the ventral midline activation of the Shh floor plate enhancer, whereas the Tbx of the CNS is dependent on an inductive signaling process site was required for repression in regions of the CNS mediated by the secreted protein Sonic hedgehog (Shh). To where Shh is not normally expressed. We further show that understand molecularly how floor plate induction proceeds Shh enhancer activity was detected in the mouse node from we identified a Shh-responsive regulatory element that where the floor plate and notochord precursors derive. Shh directs transgene reporter expression to the ventral midline reporter expression was restricted to the ventral of the CNS and notochord in a Shh-like manner and (mesodermal) layer of the node in a pattern similar to characterized critical cis-acting sequences regulating this endogenous Shh. X-gal-positive cells emerging from the element. Cross-species comparisons narrowed the activity node were only detected in the notochord lineage, of the Shh floor plate enhancer to an 88-bp sequence within suggesting that the floor plate and notochord arise from intron 2 of Shh that included highly conserved binding sites distinct precursors in the mouse node. -
Combinatorial Signals from the Neural Tube, Floor Plate and Notochord
Development 121, 651-660 (1995) 651 Printed in Great Britain © The Company of Biologists Limited 1995 Combinatorial signals from the neural tube, floor plate and notochord induce myogenic bHLH gene expression in the somite Andrea E. Münsterberg and Andrew B. Lassar Department of Biological Chemistry and Molecular Pharmacology, Harvard Medical School, 240 Longwood Avenue, Boston, MA 02115, USA SUMMARY The neural tube, floor plate and notochord are axial tissues plate/notochord, while in more rostral somites (stages IV- in the vertebrate embryo which have been demonstrated to IX) the neural tube without the floor plate/notochord is suf- play a role in somite morphogenesis. Using in vitro co- ficient. By recombining somites and neural tubes from culture of tissue explants, we have monitored inductive different axial levels of the embryo, we have found that a interactions of these axial tissues with the adjacent somitic second signal is necessary to promote competence of the mesoderm in chick embryos. We have found that signals somite to respond to inducing signals from the neural tube. from the neural tube and floor plate/notochord are Thus, we propose that at least two signals from axial tissues necessary for expression of the myogenic bHLH regulators work in combination to induce myogenic bHLH gene MyoD, Myf5 and myogenin in the somite. Eventually expression; one signal derives from the floor somitic expression of the myogenic bHLH genes is main- plate/notochord and the other signal derives from regions tained in the absence of the axial tissues. In organ culture, of the neural tube other than the floor plate. -
Neurulation Neuroanatomy > Neuroembryology > Neuroembryology
Neurulation Neuroanatomy > Neuroembryology > Neuroembryology NEURULATION SUMMARY NEURULATION Definiton • The process of neurulation involves the formation of the neural plate and the folding of the neural plate into the neural tube. Key Points • The notochord induces the overlying ectoderm to develop into the neural plate. • The neural plate folds into the neural tube and as it closes, the neural crests are pinched off. • The neural tube derives the central nervous system (the brain and spinal cord). • The neural crest cells derive the peripheral nervous system (eg, ganglion cells and Schwann cells) and also select other cell types (eg, melaoncytes). THE DEVELOPING EMBRYO Trilaminar germ disc Three layers of the trilaminar germ disc. • Ectoderm (and amniotic cavity) • Mesoderm • Endoderm (and yolk sac) THE NOTOCHORD The prochordal knot 1 / 7 • A strand of cells that extends toward the cranial end of the prochordal knot. - The prochordal knot lies within the mesoderm (in between the ectoderm and endoderm). ASSOCIATED EMBRYONIC STRUCTURES Key associated embryonic structures: • The primitive streak exists within the ectodermal layer of the germ disc; it dimples along the embryonic disc. • The primitive node (aka primitive knot, Hensen's node) lies at the cranial end of the primitive streak. • The prochordal knot lies farther cranially. NOTOCHORD DEVELOPMENT • The notochord develops cranially, (towards the head of the embryo) and because it is blocked at the prochordal plate, it also develops caudally (towards the tail of the embryo) as the primitive streak regresses. There are multiple stages of notochord development, which we omit, here, for simplicity. Key notochord actions: • Forms the embryonic central axis, • Induces neural plate formation, • Establishes the central column of the spine and then degenerates to become the nucleus pulposus of the intervertebral discs. -
Specifying Neurons and Circuits for Limb Motor Control
Specifying neurons and circuits for limb motor control Alana I. Mendelsohn Submitted in partial fulfillment of the requirements for the degree of Doctor of Philosophy under the Executive Committee of the Graduate School of Arts and Sciences COLUMBIA UNIVERSITY 2016 ©2016 Alana I. Mendelsohn All rights reserved Abstract Specifying neurons and circuits for limb motor control Alana I. Mendelsohn The emergence of limbs in vertebrates represents a significant evolutionary innovation. Limbs facilitate diverse motor behaviors, yet require spinal networks that can coordinate the activities of many individual muscles within the limb. Here I describe several efforts to characterize the specification of spinal motor neurons and assembly of spinal circuits in higher vertebrates. I discuss the formation of selective presynaptic sensory inputs to motor pools, a process which has long been thought to occur in an activity-independent manner. I demonstrate an as yet unappreciated role of activity-dependent refinement in patterning the set of sensory-motor connections that link motor pools with synergist function. I also explore the genetic specification of motor pools that project to defined muscle targets. I show that the motor pools that control digits engage distinct developmental genetic programs which reflect underlying differences in Hox and retinoic acid signaling. The divergent mechanisms underlying the specification of digit- innervating motor neurons may reflect the unique status of digit control in the evolution of motor behavior. Table of