Community Assembly of Benthic Invertebrates on Island-Like
Total Page:16
File Type:pdf, Size:1020Kb
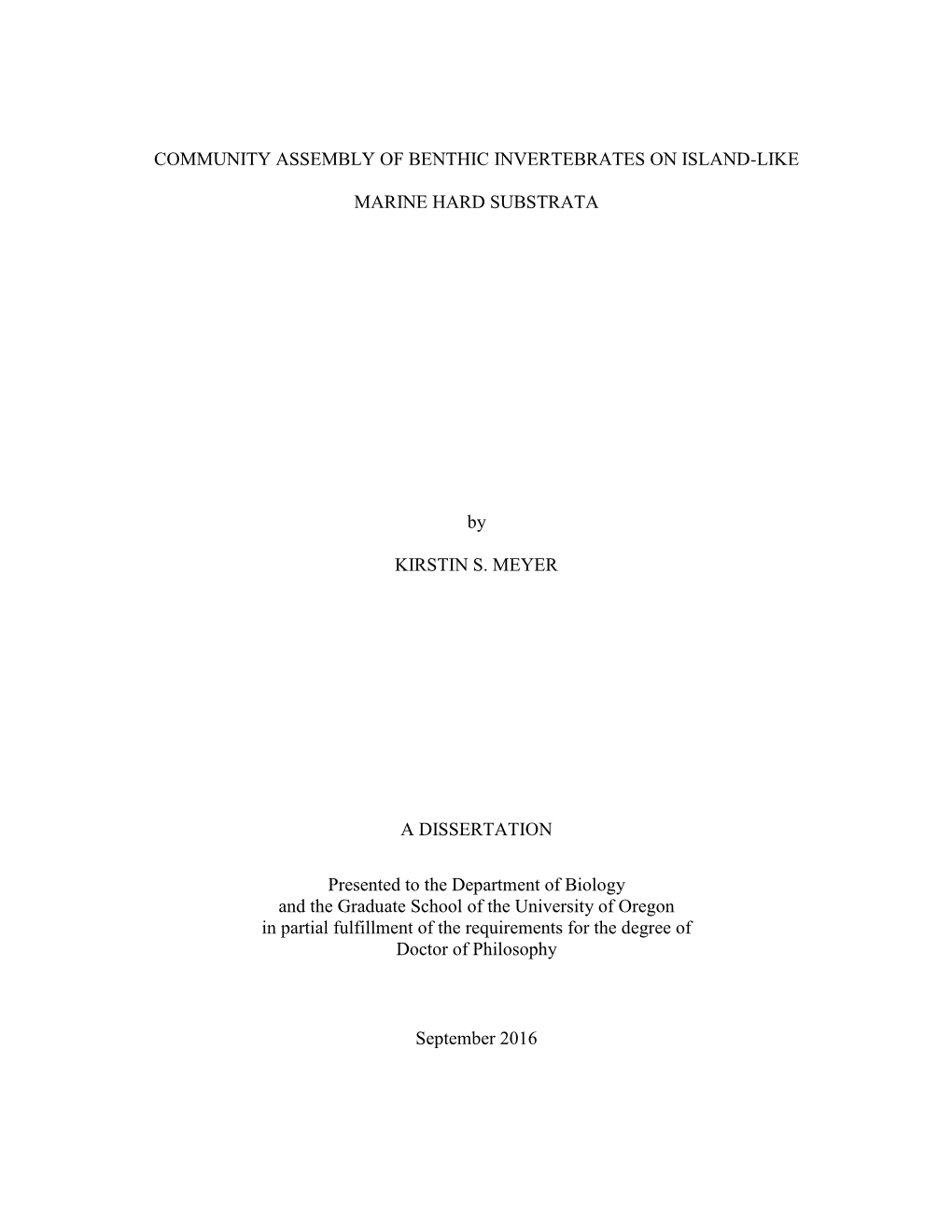
Load more
Recommended publications
-
Download PDF Version
MarLIN Marine Information Network Information on the species and habitats around the coasts and sea of the British Isles Foliose seaweeds and coralline crusts in surge gully entrances MarLIN – Marine Life Information Network Marine Evidence–based Sensitivity Assessment (MarESA) Review Dr Heidi Tillin 2015-11-30 A report from: The Marine Life Information Network, Marine Biological Association of the United Kingdom. Please note. This MarESA report is a dated version of the online review. Please refer to the website for the most up-to-date version [https://www.marlin.ac.uk/habitats/detail/31]. All terms and the MarESA methodology are outlined on the website (https://www.marlin.ac.uk) This review can be cited as: Tillin, H.M. 2015. Foliose seaweeds and coralline crusts in surge gully entrances. In Tyler-Walters H. and Hiscock K. (eds) Marine Life Information Network: Biology and Sensitivity Key Information Reviews, [on- line]. Plymouth: Marine Biological Association of the United Kingdom. DOI https://dx.doi.org/10.17031/marlinhab.31.1 The information (TEXT ONLY) provided by the Marine Life Information Network (MarLIN) is licensed under a Creative Commons Attribution-Non-Commercial-Share Alike 2.0 UK: England & Wales License. Note that images and other media featured on this page are each governed by their own terms and conditions and they may or may not be available for reuse. Permissions beyond the scope of this license are available here. Based on a work at www.marlin.ac.uk (page left blank) Date: 2015-11-30 Foliose seaweeds and coralline -
Does Oxygen Limit Thermal Tolerance in Arthropods? a Critical Review of Current Evidence
Comparative Biochemistry and Physiology, Part A 192 (2016) 64–78 Contents lists available at ScienceDirect Comparative Biochemistry and Physiology, Part A journal homepage: www.elsevier.com/locate/cbpa Review Does oxygen limit thermal tolerance in arthropods? A critical review of current evidence Wilco C.E.P. Verberk a,⁎, Johannes Overgaard b,RasmusErnb,MarkBayleyb,TobiasWangb, Leigh Boardman c,JohnS.Terblanchec a Department of Animal Ecology and Ecophysiology, Radboud University Nijmegen, Toernooiveld 1, 6525 ED Nijmegen, The Netherlands b Zoophysiology, Department of Bioscience, Aarhus University, C.F. Møllers Allé 3, Building 1131, DK-8000 Aarhus, Denmark c Department of Conservation Ecology and Entomology, Centre for Invasion Biology, Stellenbosch University, South Africa article info abstract Article history: Over the last decade, numerous studies have investigated the role of oxygen in setting thermal tolerance in aquatic an- Received 17 August 2015 imals, and there has been particular focus on arthropods. Arthropods comprise one of the most species-rich taxonomic Received in revised form 14 October 2015 groups on Earth, and display great diversity in the modes of ventilation, circulation, blood oxygen transport, with rep- Accepted 20 October 2015 resentatives living both in water (mainly crustaceans) and on land (mainly insects). The oxygen and capacity limita- Available online 24 October 2015 tion of thermal tolerance (OCLTT) hypothesis proposes that the temperature dependent performance curve of animals Keywords: is shaped by the capacity for oxygen delivery in relation to oxygen demand. If correct, oxygen limitation could provide OCLTT a mechanistic framework to understand and predict both current and future impacts of rapidly changing climate. Respiration physiology In arthropods, most studies testing the OCLTT hypothesis have considered tolerance to thermal extremes. -
Marine Biodiversity of the Northern and Yorke Peninsula NRM Region
Marine Environment and Ecology Benthic Ecology Subprogram Marine Biodiversity of the Northern and Yorke Peninsula NRM Region SARDI Publication No. F2009/000531-1 SARDI Research Report series No. 415 Keith Rowling, Shirley Sorokin, Leonardo Mantilla and David Currie SARDI Aquatic Sciences PO Box 120 Henley Beach SA 5022 December 2009 Prepared for the Department for Environment and Heritage 1 Marine Biodiversity of the Northern and Yorke Peninsula NRM Region Keith Rowling, Shirley Sorokin, Leonardo Mantilla and David Currie December 2009 SARDI Publication No. F2009/000531-1 SARDI Research Report Series No. 415 Prepared for the Department for Environment and Heritage 2 This Publication may be cited as: Rowling, K.P., Sorokin, S.J., Mantilla, L. & Currie, D.R. (2009) Marine Biodiversity of the Northern and Yorke Peninsula NRM Region. South Australian Research and Development Institute (Aquatic Sciences), Adelaide. SARDI Publication No. F2009/000531-1. South Australian Research and Development Institute SARDI Aquatic Sciences 2 Hamra Avenue West Beach SA 5024 Telephone: (08) 8207 5400 Facsimile: (08) 8207 5406 http://www.sardi.sa.gov.au DISCLAIMER The authors warrant that they have taken all reasonable care in producing this report. The report has been through the SARDI internal review process, and has been formally approved for release by the Chief of Division. Although all reasonable efforts have been made to ensure quality, SARDI does not warrant that the information in this report is free from errors or omissions. SARDI does not accept any liability for the contents of this report or for any consequences arising from its use or any reliance placed upon it. -
Chemists with No Backbones
Medicines from the Deep Sea: Exploration of the Gulf of Mexico Chemists with No Backbones FOCUS TEACHING TIME Benthic invertebrates that produce pharmacologi- One or two 45-minute class periods, plus time for cally-active substances student research GRADE LEVEL SEATING ARRANGEMENT 5-6 (Life Science) Classroom style, or groups of 2-3 students FOCUS QUESTION MAXIMUM NUMBER OF STUDENTS What groups of marine organisms produce sub- 30 stances that may be helpful in treating human dis- eases? KEY WORDS Cardiovascular disease LEARNING OBJECTIVES Cancer Students will be able to identify at least three Arthritis groups of benthic invertebrates that are known to Natural products produce pharmacologically-active compounds. Sponge Tunicate Students will be able to describe why pharmaco- Ascidian logically-active compounds derived from benthic Bryozoan invertebrates may be important in treating human Octocorals diseases. BACKGROUND INFORMATION Students will be able to infer why sessile marine Despite the many advances of modern medicine, invertebrates appear to be promising sources of disease is still the leading cause of death in the new drugs. United States. Cardiovascular disease and cancer together account for more than 1.5 million deaths MATERIALS annually (40% and 25% of all deaths, respectively). Marker board, blackboard, or overhead projector In addition, one in six Americans have some form with transparencies for group discussions of arthritis, and hospitalized patients are increas- ingly threatened by infections that are resistant to AUDIO/VISUAL MATERIALS conventional antibiotics. The cost of these diseases None is staggering: $285 billion per year for cardiovas- cular disease; $107 billion per year for cancer; $65 billion per year for arthritis. -
OCS Study BOEM 2017-024
OCS Study BOEM 2017-024 Deepwater Reconnaissance of Potentially Sensitive Biological Features Surrounding Shelf-Edge Topographical Banks in the Northern Gulf of Mexico U.S. Department of the Interior Bureau of Ocean Energy Management Gulf of Mexico OCS Region OCS Study BOEM 2017-024 Deepwater Reconnaissance of Potentially Sensitive Biological Features Surrounding Shelf-Edge Topographical Banks in the Northern Gulf of Mexico Author Paul Sammarco Prepared under BOEM Contract M11AC00005 by Louisiana Universities Marine Consortium 8124 Highway 56 Baton Rouge, LA 70344-2110 Published by U.S. Department of the Interior New Orleans, LA Bureau of Ocean Energy Management February 2017 Gulf of Mexico OCS Region DISCLAIMER This report was prepared under contract between the Bureau of Ocean Energy Management (BOEM) and Louisiana Universities Marine Consortium (LUMCON). This report has been technically reviewed by BOEM, and it has been approved for publication. Approval does not necessarily signify that the contents reflect the views and policies of BOEM, nor does mention of trade names or commercial products constitute endorsement or recommendation for use. REPORT AVAILABILITY To download a PDF file of this Gulf of Mexico OCS Region report, go to the U.S. Department of the Interior, Bureau of Ocean Energy Management, Environmental Studies Program Information System website and search on OCS Study BOEM 2017-024. This report can be viewed at select Federal Depository Libraries. It can also be obtained from the National Technical Information Service; the contact information is below. U.S. Department of Commerce National Technical Information Service 5301 Shawnee Rd. Springfield, Virginia 22312 Phone: (703) 605-6000, 1(800)553-6847 Fax: (703) 605-6900 Website: http://www.ntis.gov/ CITATION Sammarco, Paul W. -
Marlin Marine Information Network Information on the Species and Habitats Around the Coasts and Sea of the British Isles
MarLIN Marine Information Network Information on the species and habitats around the coasts and sea of the British Isles Novocrania anomala and Protanthea simplex on sheltered circalittoral rock MarLIN – Marine Life Information Network Marine Evidence–based Sensitivity Assessment (MarESA) Review John Readman & Angus Jackson 2016-03-31 A report from: The Marine Life Information Network, Marine Biological Association of the United Kingdom. Please note. This MarESA report is a dated version of the online review. Please refer to the website for the most up-to-date version [https://www.marlin.ac.uk/habitats/detail/5]. All terms and the MarESA methodology are outlined on the website (https://www.marlin.ac.uk) This review can be cited as: Readman, J.A.J. & Jackson, A. 2016. [Novocrania anomala] and [Protanthea simplex] on sheltered circalittoral rock. In Tyler-Walters H. and Hiscock K. (eds) Marine Life Information Network: Biology and Sensitivity Key Information Reviews, [on-line]. Plymouth: Marine Biological Association of the United Kingdom. DOI https://dx.doi.org/10.17031/marlinhab.5.1 The information (TEXT ONLY) provided by the Marine Life Information Network (MarLIN) is licensed under a Creative Commons Attribution-Non-Commercial-Share Alike 2.0 UK: England & Wales License. Note that images and other media featured on this page are each governed by their own terms and conditions and they may or may not be available for reuse. Permissions beyond the scope of this license are available here. Based on a work at www.marlin.ac.uk (page left blank) Date: 2016-03-31 Novocrania anomala and Protanthea simplex on sheltered circalittoral rock - Marine Life Information Network Circalittoral cliff face with dense brachiopods Neocrania anomala and Terebratulina retusa, the anemone Protanthea simplex and the ascidian Ciona intestinalis. -
Solomon Islands Marine Life Information on Biology and Management of Marine Resources
Solomon Islands Marine Life Information on biology and management of marine resources Simon Albert Ian Tibbetts, James Udy Solomon Islands Marine Life Introduction . 1 Marine life . .3 . Marine plants ................................................................................... 4 Thank you to the many people that have contributed to this book and motivated its production. It Seagrass . 5 is a collaborative effort drawing on the experience and knowledge of many individuals. This book Marine algae . .7 was completed as part of a project funded by the John D and Catherine T MacArthur Foundation Mangroves . 10 in Marovo Lagoon from 2004 to 2013 with additional support through an AusAID funded community based adaptation project led by The Nature Conservancy. Marine invertebrates ....................................................................... 13 Corals . 18 Photographs: Simon Albert, Fred Olivier, Chris Roelfsema, Anthony Plummer (www.anthonyplummer. Bêche-de-mer . 21 com), Grant Kelly, Norm Duke, Corey Howell, Morgan Jimuru, Kate Moore, Joelle Albert, John Read, Katherine Moseby, Lisa Choquette, Simon Foale, Uepi Island Resort and Nate Henry. Crown of thorns starfish . 24 Cover art: Steven Daefoni (artist), funded by GEF/IWP Fish ............................................................................................ 26 Cover photos: Anthony Plummer (www.anthonyplummer.com) and Fred Olivier (far right). Turtles ........................................................................................... 30 Text: Simon Albert, -
Marlin Marine Information Network Information on the Species and Habitats Around the Coasts and Sea of the British Isles
MarLIN Marine Information Network Information on the species and habitats around the coasts and sea of the British Isles Bloody Henry starfish (Henricia oculata) MarLIN – Marine Life Information Network Biology and Sensitivity Key Information Review Angus Jackson 2008-04-24 A report from: The Marine Life Information Network, Marine Biological Association of the United Kingdom. Please note. This MarESA report is a dated version of the online review. Please refer to the website for the most up-to-date version [https://www.marlin.ac.uk/species/detail/1131]. All terms and the MarESA methodology are outlined on the website (https://www.marlin.ac.uk) This review can be cited as: Jackson, A. 2008. Henricia oculata Bloody Henry starfish. In Tyler-Walters H. and Hiscock K. (eds) Marine Life Information Network: Biology and Sensitivity Key Information Reviews, [on-line]. Plymouth: Marine Biological Association of the United Kingdom. DOI https://dx.doi.org/10.17031/marlinsp.1131.1 The information (TEXT ONLY) provided by the Marine Life Information Network (MarLIN) is licensed under a Creative Commons Attribution-Non-Commercial-Share Alike 2.0 UK: England & Wales License. Note that images and other media featured on this page are each governed by their own terms and conditions and they may or may not be available for reuse. Permissions beyond the scope of this license are available here. Based on a work at www.marlin.ac.uk (page left blank) Date: 2008-04-24 Bloody Henry starfish (Henricia oculata) - Marine Life Information Network See online review for distribution map Henricia oculata. Distribution data supplied by the Ocean Photographer: Keith Hiscock Biogeographic Information System (OBIS). -
Deep-Sea Fauna of the European Seas: an Annotated Species Check-List Of
Invertebrate Zoology, 2014, 11(1): 134–155 © INVERTEBRATE ZOOLOGY, 2014 Deep-sea fauna of European seas: An annotated species check-list of benthic invertebrates living deeper than 2000 m in the seas bordering Europe. Gastropoda Alexander V. Sysoev Zoological Museum, Moscow State University, Bol’shaya Nikitskaya ul., 6, Moscow, 125009, Russia. E-mail: [email protected] ABSTRACT: An annotated check-list is given of Gastropoda species occurring deeper than 2000 m in the seas bordering Europe. The check-list is based on published data. The check- list includes 221 species. For each species data on localities in European seas and general species distribution are provided. Station data are presented separately in the present thematic issue. How to cite this article: Sysoev A.V. 2014. Deep-sea fauna of European seas: An annotated species check-list of benthic invertebrates living deeper than 2000 m in the seas bordering Europe. Gastropoda // Invert. Zool. Vol.11. No.1. P.134–155. KEY WORDS: deep-sea fauna, European seas, Gastropoda. Глубоководная фауна европейских морей: аннотированный список видов донных беспозвоночных, обитающих глубже 2000 м в морях, окружающих Европу. Gastropoda А.В. Сысоев Зоологический музей МГУ им. М.В. Ломоносова, ул. Большая Никитская, 6, Москва 125009, Россия. E-mail: [email protected] РЕЗЮМЕ: Приводится аннотированный список видов Gastropoda, обитающих глуб- же 2000 м в морях, окружающих Европу. Список основан на опубликованных данных. Список насчитывает 221 вид. Для каждого вида приведены данные о нахождениях в европейских морях и сведения о распространении. Данные о станци- ях приводятся в отдельном разделе настоящего тематического выпуска. Как цитировать эту статью: Sysoev A.V. -
Calypso LNG Deepwater Port Project, Florida: Marine Benthic Video Survey Charles Messing Nova Southeastern University Oceanographic Center, [email protected]
Nova Southeastern University NSUWorks Marine & Environmental Sciences Faculty Reports Department of Marine and Environmental Sciences 6-12-2006 Calypso LNG Deepwater Port Project, Florida: Marine Benthic Video Survey Charles Messing Nova Southeastern University Oceanographic Center, [email protected] Brian K. Walker Nova Southeastern University Oceanographic Center, [email protected] Richard E. Dodge Nova Southeastern University Oceanographic Center, [email protected] John K. Reed Harbor Branch Oceanographic Institution Sandra Brooke Florida Fish and Wildlife Research Institute Find out more information about Nova Southeastern University and the Halmos College of Natural Sciences and Oceanography. Follow this and additional works at: https://nsuworks.nova.edu/occ_facreports Part of the Marine Biology Commons, and the Oceanography and Atmospheric Sciences and Meteorology Commons NSUWorks Citation Charles Messing, Brian K. Walker, Richard E. Dodge, John K. Reed, and Sandra Brooke. 2006. Calypso LNG Deepwater Port Project, Florida: Marine Benthic Video Survey : 1 -61. https://nsuworks.nova.edu/occ_facreports/77. This Article is brought to you for free and open access by the Department of Marine and Environmental Sciences at NSUWorks. It has been accepted for inclusion in Marine & Environmental Sciences Faculty Reports by an authorized administrator of NSUWorks. For more information, please contact [email protected]. Calypso LNG Deepwater Port Project, Florida Marine Benthic Video Survey FINAL REPORT 12 June 2006 Submitted to: Ecology and Environment, Inc. & SUEZ Energy North America, Inc. Submitted by: Charles G. Messing, Ph.D., Brian K. Walker, M.S. and Richard E. Dodge, Ph.D. National Coral Reef Institute, Nova Southeastern University Oceanographic Center, 8000 North Ocean Drive, Dania Beach, FL 33004 John Reed, M.S., Harbor Branch Oceanographic Institution 5600 U.S. -
Auswirkungen Der Ozeanversauerung Auf Die Große Seespinne Hyas Araneus: Molekulare Mechanismen Und Plastizität
Auswirkungen der Ozeanversauerung auf die große Seespinne Hyas araneus: Molekulare Mechanismen und Plastizität Dissertation zur Erlangung des akademischen Grades – Dr. rer. nat. – Dem Fachbereich 2 Biologie/Chemie der Universität Bremen vorgelegt von Lars Harms Diplombiologe Bremen 2014 Der Einband enthält Illustrationen von Allen (1967) und Hugo Ahlenius (UNEP/GRID-Arendal) Gutachter: 1. Gutachter: Professor Dr. H.-O. Pörtner Alfred-Wegener-Institut – Helmholtz-Zentrum für Polar- und Meeresforschung Am Handelshafen 12, 27570 Bremerhaven 2. Gutachter: Associate Professor Dr. L. Tomanek California Polytechnic State University San Luis Obispo, California 93407, United States of America Inhaltsverzeichnis Inhaltsverzeichnis Abkürzungsverzeichnis III Zusammenfassung V Abstract VII 1 Einleitung 1 1.1 Das Projekt BIOACID 1 1.2 Einfluss von Ozeanversauerung auf marine Crustaceen 3 1.3 Kieme als Modelgewebe 6 1.4 Hyas araneus als Modelorganismus 8 1.5 Konzept und Fragestellung 11 2 Material und Methoden 13 2.1 Versuchstiere 13 2.2 Experimenteller Aufbau und Bestimmung der Meerwasserparameter 13 2.3 Probennahme und Hämolymph-Analytik 14 2.4 Molekulare Analytik 15 RNA Extraktion und Sequenzierung 15 Assemblierung, funktionelle Annotation und Sequenzalignierung 16 Quantitative Real-Time PCR 17 Protein-Aufbereitung 19 Zweidimensionale Gel-Elektrophorese und Gelanalyse 19 Massenspektrometrie 20 2.5 Statistik 21 Vergleichende Analyse 21 Transkriptomik 22 Proteomik 22 3 Publikationen 23 Publikation I 25 "Characterization and analysis -
FAU Institutional Repository
FAU Institutional Repository http://purl.fcla.edu/fau/fauir This paper was submitted by the faculty of FAU’s Harbor Branch Oceanographic Institute. Notice: ©1990 The Bailey-Matthews Shell Museum. This author manuscript appears courtesy of The Nautilus, a peer-reviewed, not-for-profit quarterly published by the non-profit organization The Bailey-Matthews Shell Museum. The published version is available at http://shellmuseum.org/nautilus/index.html and may be cited as: Harasewyeh, M. G. (1990). Studies on bathyal and abyssal buccinidae (Gastropoda: Neogastropoda): 1. Metula fusiformis Clench and Aguayo, 1941. The Nautilus, 104(4), 120-129. o THE NAUTI LUS 104(4):120-129, 1990 Page 120 Studies on Bathyal and Abyssal Buccinidae (Gastropoda: Neogastropoda): 1. Metula fusiformis Clench and Aguayo, 1941 M. G. Harasewych Department of Invertebrate Zoology National Museum of Natura l History Smithsonian Institution Washington , DC 20560, USA ABSTRACT fact that the vast majority of taxa are based exclusively on features of the shell and operculum, supplemented Based on the morphology of the radu la and shell, Metula [u occasionally by observations on radu lar morphology. sifo rmis Clench & Aguayo, 1941 is transferred to the predom inantl y Indo-w estern Pacific genus Manaria . This species occurs Shells of Buccinid ae tend to be simple, and offer few in upper continental slope communities (183- 578 m) of the readily discernible morphological characters. These are Caribbean Sea and the northern coast of South America . The subject to convergence, especia lly in polar regions and holotype was collected dead in 2,633 rn, well below the depth the deep sea, where effects of habitat on shell form are inhabited by this species.