G GE Aviation the Aircraft Engine Design Project Fundamentals
Total Page:16
File Type:pdf, Size:1020Kb
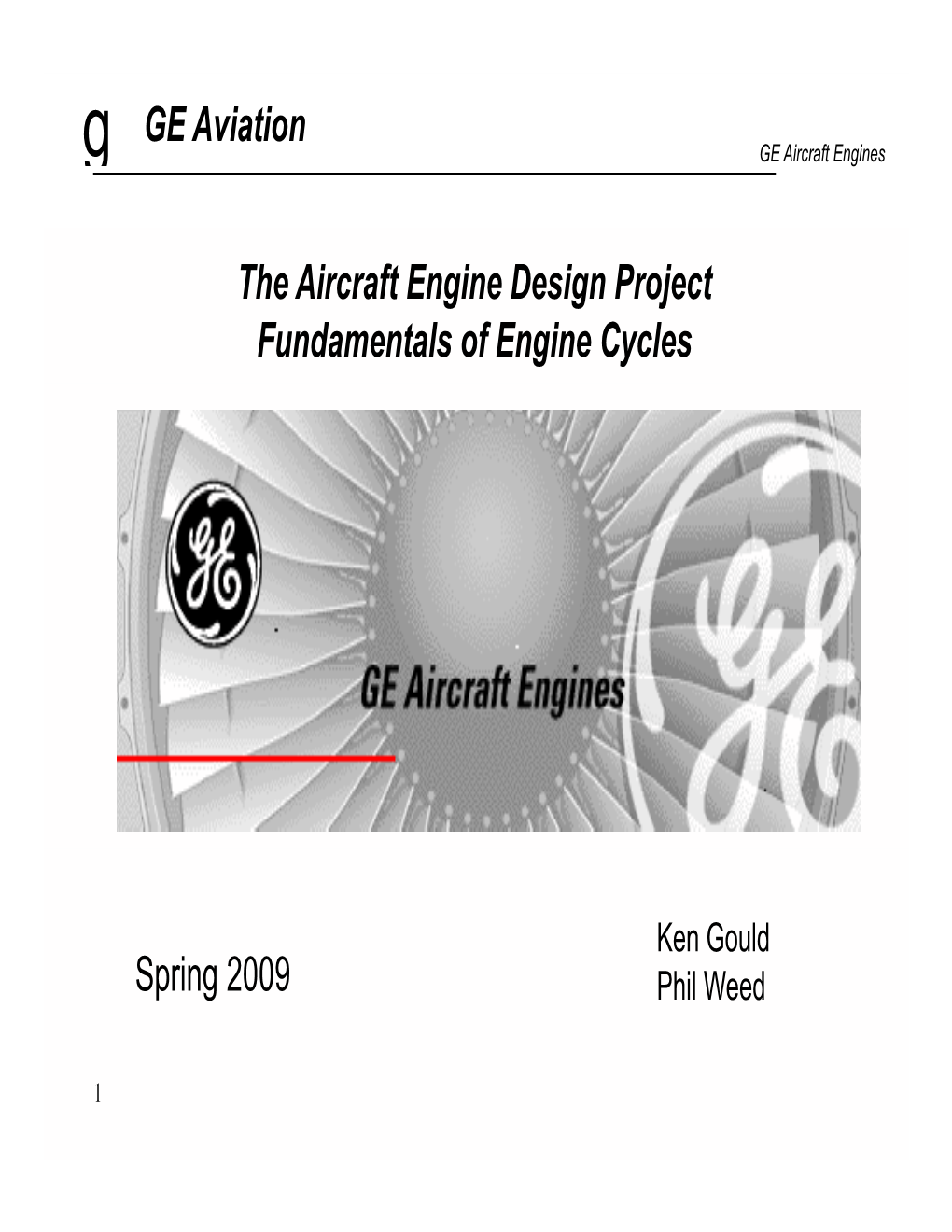
Load more
Recommended publications
-
Aircraft Engine Performance Study Using Flight Data Recorder Archives
Aircraft Engine Performance Study Using Flight Data Recorder Archives Yashovardhan S. Chati∗ and Hamsa Balakrishnan y Massachusetts Institute of Technology, Cambridge, Massachusetts, 02139, USA Aircraft emissions are a significant source of pollution and are closely related to engine fuel burn. The onboard Flight Data Recorder (FDR) is an accurate source of information as it logs operational aircraft data in situ. The main objective of this paper is the visualization and exploration of data from the FDR. The Airbus A330 - 223 is used to study the variation of normalized engine performance parameters with the altitude profile in all the phases of flight. A turbofan performance analysis model is employed to calculate the theoretical thrust and it is shown to be a good qualitative match to the FDR reported thrust. The operational thrust settings and the times in mode are found to differ significantly from the ICAO standard values in the LTO cycle. This difference can lead to errors in the calculation of aircraft emission inventories. This paper is the first step towards the accurate estimation of engine performance and emissions for different aircraft and engine types, given the trajectory of an aircraft. I. Introduction Aircraft emissions depend on engine characteristics, particularly on the fuel flow rate and the thrust. It is therefore, important to accurately assess engine performance and operational fuel burn. Traditionally, the estimation of fuel burn and emissions has been done using the ICAO Aircraft Engine Emissions Databank1. However, this method is approximate and the results have been shown to deviate from the measured values of emissions from aircraft in operation2,3. -
Comparison of Helicopter Turboshaft Engines
Comparison of Helicopter Turboshaft Engines John Schenderlein1, and Tyler Clayton2 University of Colorado, Boulder, CO, 80304 Although they garnish less attention than their flashy jet cousins, turboshaft engines hold a specialized niche in the aviation industry. Built to be compact, efficient, and powerful, turboshafts have made modern helicopters and the feats they accomplish possible. First implemented in the 1950s, turboshaft geometry has gone largely unchanged, but advances in materials and axial flow technology have continued to drive higher power and efficiency from today's turboshafts. Similarly to the turbojet and fan industry, there are only a handful of big players in the market. The usual suspects - Pratt & Whitney, General Electric, and Rolls-Royce - have taken over most of the industry, but lesser known companies like Lycoming and Turbomeca still hold a footing in the Turboshaft world. Nomenclature shp = Shaft Horsepower SFC = Specific Fuel Consumption FPT = Free Power Turbine HPT = High Power Turbine Introduction & Background Turboshaft engines are very similar to a turboprop engine; in fact many turboshaft engines were created by modifying existing turboprop engines to fit the needs of the rotorcraft they propel. The most common use of turboshaft engines is in scenarios where high power and reliability are required within a small envelope of requirements for size and weight. Most helicopter, marine, and auxiliary power units applications take advantage of turboshaft configurations. In fact, the turboshaft plays a workhorse role in the aviation industry as much as it is does for industrial power generation. While conventional turbine jet propulsion is achieved through thrust generated by a hot and fast exhaust stream, turboshaft engines creates shaft power that drives one or more rotors on the vehicle. -
Wankel Powered Time to Climb World Record Attempt
Wankel powered time to climb world record attempt Presentation at Aero Expo, Friedrichshafen, Germany April 19th, 2018 by Paul Lamar Several years ago an American rotor head by the name of Russ MacFarlane living in Newcastle Australia decided to build a Mazda rotary powered world record attempt time to climb aircraft. Russ was a long time subscriber to the Rotary Engine News Letter. A used flying Harmon Rocket home built aircraft was purchased and the Lycoming engine and instruments were removed and sold. The aircraft is very similar to a Van's RV4 with longer landing gear and shorter wings. Dan Grey, owner of Aviation FX in Santa Paula California was chosen to finish the project and get it flying. I was the project engineer. Dan is a 787 captain for UAL. The Wankel rotary has a much better power to weight ratio and power to size ratio than any automotive piston engine. It is also far more robust and will withstand ungodly amounts of turbo boost with out structural failure. Up to 100 inches of Mercury manifold pressure is possible. That translates to almost 1000 HP for an all aluminum turbo two rotor weighing less than 200 pounds. That is about four HP per pound of engine weight. Most aircraft engines are about one HP per pound of weight or worse. Any piston engine operating at aircraft power levels has a limited life. That is the reason for a TBO. The moving parts are magnafluxed for cracks at TBO. The cracks are caused by reversing stress on the crankshaft, connecting rods, pistons and valve parts. -
Siemens Eaircraft Overview
eAircraft: Hybrid-elektrische Antriebe für Luftfahrzeuge Dr. Frank Anton, Siemens AG, Corporate Technology 14. Tag der Deutschen Luft- und Raumfahrtregionen, Potsdam, 10. September 2019 © Siemens AG 2019 siemens.com We develop hybrid electric propulsion systems for aircraft Hybrid-electric propulsion is a scalable technology eVTOL potentially large number economy of scale Enabler to reduce total cost of ownership and envinronmental impact: Hybrid electric propulsion Useful range Decreased fuel flow & emission Separation of power generation and thrust generation Silent propulsion Distributed propulsion Increased aerodynamic efficiency Vectorized thrust eSTOL, eVTOL Page 2 Dr. Frank Anton, Siemens AG eAircraft September 10th, 2019 Unrestricted © Siemens AG 2019. All rights reserved The eAircraft portfolio has been designed to meet aerospace requirements and is now on the way to industrialization & certification The eAircraft portfolio has been shaped by close collaboration with partners such as Airbus. In the lower power classes, the systems have already been tested in flight and are being installed in first commercial applications. In the high power classes, a 2 MW lab demonstrator is currently awaiting test results and a design of a 10 MW generator based on superconducting technology exists as digital twin. Page 3 Dr. Frank Anton, Siemens AG eAircraft September 10th, 2019 Unrestricted © Siemens AG 2019. All rights reserved Our core portfolio – electric propulsion units (EPU) for applications with high power/weight requirements Condition-based Services System Integration Controller Fuel Battery Cell DC/DC Inverter Power Signal AC/DC DC/AC Generator Distri- Distri- Motor G Inverter Inverter bution bution Turbine/ICE Siemens Trading item Page 4 Dr. Frank Anton, Siemens AG eAircraft September 10th, 2019 Unrestricted © Siemens AG 2019. -
Method for Determining the Applicability of an Air Turbine for Operation in a Gas Turbine Engine Launch System
Method for Determining the Applicability of an Air Turbine for Operation in a Gas Turbine Engine Launch System Vasilii Zubanov1a, Grigorii Popov1b, Igor Egorov2, Evgenii Marchukov2 and Yulia Novikova1 1Samara National Research University, Samara, Russia 2Moscow Aviation Institute, Moscow, Russia Keywords: Auxiliary Power Unit, Air Turbine, Joint Operation, Gas Turbine Engine, Start-up Time, Torque. Abstract: The paper describes the methodology developed by the authors for matching the working process of the auxiliary power unit and the air turbine used when starting the engine The need for this technique is caused by an extremely small number of publications on this topic. The developed technique can be used to determine the possibility of starting a gas turbine engine, as well as to calculate its time and main parameters under all operating conditions (including in flight) and to select new auxiliary power units or an air turbine for an existing system. The developed technique considers structural, strength, operational and other limitations. The results were implemented as a computer program. NOMENCLATURE 1 INTRODUCTION GTE - gas turbine engine The start of an aircraft gas turbine engine is an APU - auxiliary power unit important mode that largely determines the safety, NGV - nozzle guide vane operational efficiency and reliability of the engine ATS - air turbo starter and the aircraft. The gas turbine engine start-up IGV - inlet guide vane system includes a whole set of different devices and - mass flow parameter units: a starter, auxiliary power unit, air and fuel - power parameter systems, automatic control system, transmission, P - pressure power supply system, ignition system, etc. For T - temperature reliable engine start-up, the operation of all the G - mass flow rate devices must be consistent with each other. -
Diesel, Spark-Ignition, and Turboprop Engines for Long-Duration Unmanned Air Flights
JOURNAL OF PROPULSION AND POWER Diesel, Spark-Ignition, and Turboprop Engines for Long-Duration Unmanned Air Flights Daniele Cirigliano,∗ Aaron M. Frisch,† Feng Liu,‡ and William A. Sirignano‡ University of California, Irvine, California 92697 DOI: 10.2514/1.B36547 Comparisons are made for propulsion systems for unmanned flights with several hundred kilowatts of propulsive power at moderate subsonic speeds up to 50 h in duration. Gas-turbine engines (turbofans and turboprops), two- and four-stroke reciprocating (diesel and spark-ignition) engines, and electric motors (with electric generation by a combustion engine) are analyzed. Thermal analyses of these engines are performed in the power range of interest. Consideration is given to two types of generic missions: 1) a mission dominated by a constant-power requirement, and 2) a mission with intermittent demand for high thrust and/or substantial auxiliary power. The weights of the propulsion system, required fuel, and total aircraft are considered. Nowadays, diesel engines for airplane applications are rarely a choice. However, this technology is shown to bea very serious competitor for long-durationunmanned air vehicle flights. The two strongest competitors are gas-turbine engines and turbocharged four-stroke diesel engines, each type driving propellers. It is shown that hybrid-electric schemes and configurations with several propellers driven by one power source are less efficient. At the 500 KW level, one gas-turbine engine driving a larger propeller is more efficient for durations up to 25 h, whereas several diesel engines driving several propellers become more efficient at longer durations. The decreasing efficiency of the gas-turbine engine with decreasing size and increasing compression ratio is a key factor. -
A High Fidelity Real-Time Simulation of a Small Turboshaft Engine
NASA Technical Memorandum 100991 "l A High Fidelity Real-Time Simulation of a Small Turboshaft Engine Mark G. Ballin = NBB-2637B |NAS_-TM- 100991) A HIGH FIDELITY EEAL-TI_E SIMULATION OF A SMALL TDRBOSHAFT ENGINE CSCL _IE [NASA) 95 p Unclas G3/08 0156166 July 1988 | | = National Aeronautics and Space Administration NASATechnicalMemorandum100991 A High Fidelity Real-Time Simulation of a Small Turboshaft Engine Mark G. Ballin, Ames Research Center, Moffett Field, California July 1988 I_JASA National Aeronautics and Space Administration Ames Research Center MoffettField,California 94035 National Aeronautics and Space Administration N/ A Ames Research Center Moffett Field, California 94035 Shelley J. Scarlch Reply to At'In of: M/S 241-13 ERRATA RE: NASA TM 100991 A High-Fidellty Real-Time Simulation of a Small Turboshaft Engine Mark G. Ballin July 1988 On p. 2, figure i, a correction should be noted. In the upper middle portion of the figure, GAS INDUCED FLAP REGRESSIVE should be CONTROL-SYSTEM-INDUCED FLAP REGRESSIVE. Yours, Publications = DRAFT A HIGH FIDELITY REAL-TIME SIMULATION OF A SMALL TURBOSHAFT ENGINE Mark G. Ball.in Ames Research Center SUMMARY A high-fidellty component-type model and real-time digital simulation of the General Electric T700-GE-700 turboshaft engine were developed for use with current generation real-time blade-dement rotor helicopter simulations. A control system model based on the specification fuel control system used in the UH-60A Black Hawk helicopter is also pre- sented. The modeling assumptions and real-time digital implementation methods particular to the simulation of small turboshaft engines are described. -
STS-1000: a High Performance Turboshaft Engine for Hybrid
AIAA 2018-2018 Engine Design Competition Sharif University of Technology STS-IDOO: A Candidate T urboshaft Engine for Hybrid Electric Medium Altitude Long Endurance Search and Rescue UAV High PowBr to WBight Low FuBI Consumption Modular 6 Compact SIGNATURE SHEET Prof. Kaveh Ghorbanian M. Reza AminiMagham Alireza Ebrahimi Faculty Advisor Project Advisor Team Leader 952166 Amir Nazemi Abolfazl Zolfaghari Hojjat Etemadianmofrad Vahid Danesh 981123 919547 964808 964807 M. Mahdi Asnaashari Saeide Kazembeigi Mahdi Jamshidiha Amirreza Saffizadeh 952842 978931 688249 937080 Copyright © 2019 by FARAS. Published by the American Institute of Aeronautics and Astronautics, Inc., with Permission Executive Summary This report proposes a turboshaft engine referred to “Sharif TurboShaft 1000 (STS-1000)” as a candidate engine to replace the baseline engine TPE331-10 for the next generation “Hybrid Electric Medium Altitude Long Endurance Search and Rescue UAV” by the year 2025. STS-1000, unlike the baseline engine, is a split single-spool turboshaft engine. The hot gas generator is a single spool with a single stage radial compressor, a reverse annular combustion chamber, and an uncooled single stage axial compressor turbine. The required shaft power is produced by a two stage axial power turbine on a separate spool which passes through the spool of the core engine and is intended to drive a power generator at the cold end of the engine. The air intake is of S-type and the exhaust duct has circular cross section. Compared to TPE331-10, STS-1000 has a higher turbine inlet temperature, a lower stage number for the air compressor, and requires less mass flow rate. -
Aircraft Technology Roadmap to 2050 | IATA
Aircraft Technology Roadmap to 2050 NOTICE DISCLAIMER. The information contained in this publication is subject to constant review in the light of changing government requirements and regulations. No subscriber or other reader should act on the basis of any such information without referring to applicable laws and regulations and/or without taking appropriate professional advice. Although every effort has been made to ensure accuracy, the International Air Transport Association shall not be held responsible for any loss or damage caused by errors, omissions, misprints or misinterpretation of the contents hereof. Furthermore, the International Air Transport Association expressly disclaims any and all liability to any person or entity, whether a purchaser of this publication or not, in respect of anything done or omitted, and the consequences of anything done or omitted, by any such person or entity in reliance on the contents of this publication. © International Air Transport Association. All Rights Reserved. No part of this publication may be reproduced, recast, reformatted or transmitted in any form by any means, electronic or mechanical, including photocopying, recording or any information storage and retrieval system, without the prior written permission from: Senior Vice President Member & External Relations International Air Transport Association 33, Route de l’Aéroport 1215 Geneva 15 Airport Switzerland Table of Contents Table of Contents .............................................................................................................................................................................................................. -
04 Propulsion
Aircraft Design Lecture 2: Aircraft Propulsion G. Dimitriadis and O. Léonard APRI0004-1, Aerospace Design Project, Lecture 4 1 Introduction • A large variety of propulsion methods have been used from the very start of the aerospace era: – No propulsion (balloons, gliders) – Muscle (mostly failed) – Steam power (mostly failed) – Piston engines and propellers – Rocket engines – Jet engines – Pulse jet engines – Ramjet – Scramjet APRI0004-1, Aerospace Design Project, Lecture 4 2 Gliding flight • People have been gliding from the- mid 18th century. The Albatross II by Jean Marie Le Bris- 1849 Otto Lillienthal , 1895 APRI0004-1, Aerospace Design Project, Lecture 4 3 Human-powered flight • Early attempts were less than successful but better results were obtained from the 1960s onwards. Gerhardt Cycleplane (1923) MIT Daedalus (1988) APRI0004-1, Aerospace Design Project, Lecture 4 4 Steam powered aircraft • Mostly dirigibles, unpiloted flying models and early aircraft Clément Ader Avion III (two 30hp steam engines, 1897) Giffard dirigible (1852) APRI0004-1, Aerospace Design Project, Lecture 4 5 Engine requirements • A good aircraft engine is characterized by: – Enough power to fulfill the mission • Take-off, climb, cruise etc. – Low weight • High weight increases the necessary lift and therefore the drag. – High efficiency • Low efficiency increases the amount fuel required and therefore the weight and therefore the drag. – High reliability – Ease of maintenance APRI0004-1, Aerospace Design Project, Lecture 4 6 Piston engines • Wright Flyer: One engine driving two counter- rotating propellers (one port one starboard) via chains. – Four in-line cylinders – Power: 12hp – Weight: 77 kg APRI0004-1, Aerospace Design Project, Lecture 4 7 Piston engine development • During the first half of the 20th century there was considerable development of piston engines. -
Aircraft Piston Engine Operation Principles and Theory
Lect-25 Aircraft Piston Engine Operation Principles and Theory 1 Prof. Bhaskar Roy, Prof. A M Pradeep, Department of Aerospace, IIT Bombay Lect-25 How an IC engine operates-1 • Each piston is inside a cylinder, into which a gas is created -- heated inside the cylinder by ignition of a fuel air mixture at high pressure (internal combustion engine). • The hot, high pressure gases expand, pushing the piston to the bottom of the cylinder (BDC) creating Power stroke. 2 Prof. Bhaskar Roy, Prof. A M Pradeep, Department of Aerospace, IIT Bombay Lect-25 How an IC engine operates-2 •The piston is returned to the cylinder top (Top Dead Centre) either by a flywheel or the power from other pistons connected to the same shaft. • In most types the "exhausted" gases are removed from the cylinder by this stroke. • This completes the four strokes of a 4-stroke engine also representing 4 legs of a cycle 3 Prof. Bhaskar Roy, Prof. A M Pradeep, Department of Aerospace, IIT Bombay Lect-25 How an IC engine operates-3 • The linear motion of the piston is converted to a rotational motion via a connecting rod and a crankshaft. • A flywheel is used to ensure continued smooth rotation (i.e. when there is no power stroke). Multiple cylinder power strokes act as a flywheel. 4 Prof. Bhaskar Roy, Prof. A M Pradeep, Department of Aerospace, IIT Bombay Lect-25 How an IC engine operates-4 •The more cylinders a reciprocating engine has, generally, the more vibration-free (smoothly) it can operate. •The aggregate power of a reciprocating engine is proportional to the volume of the combined pistons' displacement. -
Signature Redacted Signature of Author: Delartrent of Mechanical Engineering August 1987 Signature Redacted Certified By: Prof
HEAT TRANSFER AND PERFORMANCE CALCULATIONS IN A ROTARY ENGINE by RAYMOND ANTHONY STANTEN Bachelor of Science Mechanical Engineering Rensselaer Polytechnic Institute (1985) SUBMITTED IN PARTIAL FULFILLMENT OF THE REQUIREMENTS OF THE DEGREE OF MASTER OF SCIENCE IN MECHANICAL ENGINEERING at the MASSACHUSETTS INSTITUTE OF TECHNOLOGY August 1987 Copyright Massachusetts Institute of Technology, 1987 Signature redacted Signature of Author: Delartrent of Mechanical Engineering August 1987 Signature redacted Certified by: Prof. John B. Heywood, Thksis Supervisor Dept. of Mechanical Engineering Signature redacted Accepted by: Prof. Ain A. Sonin Ch ' . pt. of Mechanical Engineering ArchiveS -2- HEAT TRANSFER AND PERFORMANCE CALCULATIONS IN A ROTARY ENGINE by RAYMOND ANTHONY STANTEN Submitted to the Department of Mechanical Engineering on August 25, 1987 in partial fulfillment of the requirements for the Degree of Master of Science in Mechanical Engineering ABSTRACT The Wankel stratified-charge combustion engine is a promising future powerplant for general aviation. The advantages of the engine include low weight, high specific power density and multifuel capability without a loss in performance. Additional gains in performance may be possible if ceramics are used to insulate the rotary engine housing, thereby increasing engine wall temperatures and reducing heat transfer losses. To evaluate the feasibility of this insulation and its effect on performance, it was necessary to determine the local temperature and heat transfer profiles at specific locations within the engine. With this boundary condition information, a finite element calculation could be used to determine the temperature fluctuation and penetration depth. This data can then be used to calculate thermal stresses which are important in ceramic applications due to the high thermal resistivity and brittleness of ceramics relative to steel.