S41598-021-95881-Z.Pdf
Total Page:16
File Type:pdf, Size:1020Kb
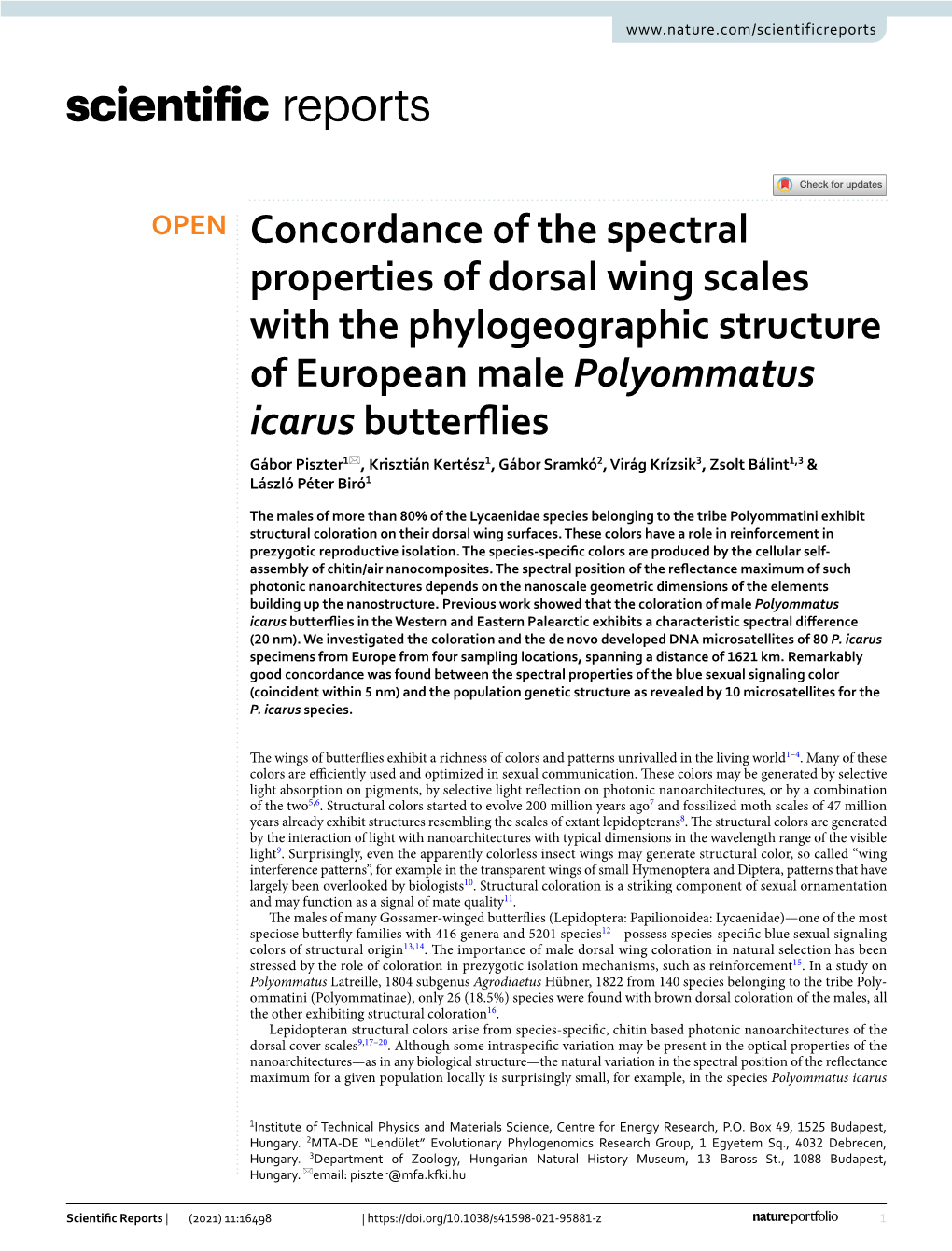
Load more
Recommended publications
-
2017, Jones Road, Near Blackhawk, RAIN (Photo: Michael Dawber)
Edited and Compiled by Rick Cavasin and Jessica E. Linton Toronto Entomologists’ Association Occasional Publication # 48-2018 European Skippers mudpuddling, July 6, 2017, Jones Road, near Blackhawk, RAIN (Photo: Michael Dawber) Dusted Skipper, April 20, 2017, Ipperwash Beach, LAMB American Snout, August 6, 2017, (Photo: Bob Yukich) Dunes Beach, PRIN (Photo: David Kaposi) ISBN: 978-0-921631-53-7 Ontario Lepidoptera 2017 Edited and Compiled by Rick Cavasin and Jessica E. Linton April 2018 Published by the Toronto Entomologists’ Association Toronto, Ontario Production by Jessica Linton TORONTO ENTOMOLOGISTS’ ASSOCIATION Board of Directors: (TEA) Antonia Guidotti: R.O.M. Representative Programs Coordinator The TEA is a non-profit educational and scientific Carolyn King: O.N. Representative organization formed to promote interest in insects, to Publicity Coordinator encourage cooperation among amateur and professional Steve LaForest: Field Trips Coordinator entomologists, to educate and inform non-entomologists about insects, entomology and related fields, to aid in the ONTARIO LEPIDOPTERA preservation of insects and their habitats and to issue Published annually by the Toronto Entomologists’ publications in support of these objectives. Association. The TEA is a registered charity (#1069095-21); all Ontario Lepidoptera 2017 donations are tax creditable. Publication date: April 2018 ISBN: 978-0-921631-53-7 Membership Information: Copyright © TEA for Authors All rights reserved. No part of this publication may be Annual dues: reproduced or used without written permission. Individual-$30 Student-free (Association finances permitting – Information on submitting records, notes and articles to beyond that, a charge of $20 will apply) Ontario Lepidoptera can be obtained by contacting: Family-$35 Jessica E. -
The European Grassland Butterfly Indicator: 1990–2011
EEA Technical report No 11/2013 The European Grassland Butterfly Indicator: 1990–2011 ISSN 1725-2237 EEA Technical report No 11/2013 The European Grassland Butterfly Indicator: 1990–2011 Cover design: EEA Cover photo © Chris van Swaay, Orangetip (Anthocharis cardamines) Layout: EEA/Pia Schmidt Copyright notice © European Environment Agency, 2013 Reproduction is authorised, provided the source is acknowledged, save where otherwise stated. Information about the European Union is available on the Internet. It can be accessed through the Europa server (www.europa.eu). Luxembourg: Publications Office of the European Union, 2013 ISBN 978-92-9213-402-0 ISSN 1725-2237 doi:10.2800/89760 REG.NO. DK-000244 European Environment Agency Kongens Nytorv 6 1050 Copenhagen K Denmark Tel.: +45 33 36 71 00 Fax: +45 33 36 71 99 Web: eea.europa.eu Enquiries: eea.europa.eu/enquiries Contents Contents Acknowledgements .................................................................................................... 6 Summary .................................................................................................................... 7 1 Introduction .......................................................................................................... 9 2 Building the European Grassland Butterfly Indicator ........................................... 12 Fieldwork .............................................................................................................. 12 Grassland butterflies ............................................................................................. -
Butterflies & Flowers of the Kackars
Butterflies and Botany of the Kackars in Turkey Greenwings holiday report 14-22 July 2018 Led by Martin Warren, Yiannis Christofides and Yasemin Konuralp White-bordered Grayling © Alan Woodward Greenwings Wildlife Holidays Tel: 01473 254658 Web: www.greenwings.co.uk Email: [email protected] ©Greenwings 2018 Introduction This was the second year of a tour to see the wonderful array of butterflies and plants in the Kaçkar mountains of north-east Turkey. These rugged mountains rise steeply from Turkey’s Black Sea coast and are an extension of the Caucasus mountains which are considered by the World Wide Fund for Nature to be a global biodiversity hotspot. The Kaçkars are thought to be the richest area for butterflies in this range, a hotspot in a hotspot with over 160 resident species. The valley of the River Çoruh lies at the heart of the Kaçkar and the centre of the trip explored its upper reaches at altitudes of 1,300—2,300m. The area consists of steep-sided valleys with dry Mediterranean vegetation, typically with dense woodland and trees in the valley bottoms interspersed with small hay-meadows. In the upper reaches these merge into alpine meadows with wet flushes and few trees. The highest mountain in the range is Kaçkar Dağı with an elevation of 3,937 metres The tour was centred around the two charming little villages of Barhal and Olgunlar, the latter being at the fur- thest end of the valley that you can reach by car. The area is very remote and only accessed by a narrow road that winds its way up the valley providing extraordinary views that change with every turn. -
7-1 1 May 2007
Volume 7 Number 1 1 May 2007 The Taxonomic Report OF THE INTERNATIONAL LEPIDOPTERA SURVEY A Description of a New Subspecies of Lycaena phlaeas (Lycaenidae: Lycaeninae) from Montana, United States, With a Comparative Study of Old and New World Populations Steve Kohler 125 Hillcrest Loop, Missoula, Montana 59803 United States of America Abstract: The Palaearctic, Oriental and Ethiopian Region subspecies of Lycaena phlaeas are briefly discussed. A more detailed account of the North American subspecies is presented, and a new subspecies, L. p. weberi, from the Sweet Grass Hills, Montana is described. The possibility that the eastern United States subspecies hypophlaeas was introduced from the Old World is discussed; however no conclusion can be reached with certainty. The relationship between Old World and New World subspecies of L. phlaeas is discussed. Evidence presented supports the treatment of New World populations as subspecies of L. phlaeas. Additional key words: Polygonaceae, Rumex acetosella, R. acetosa, R. crispus, Oxyria digyna. INTRODUCTION Lycaena phlaeas (Linnaeus, 1761) is a widespread species with subspecies in Europe, North Africa, Arabia, northern Asia, Japan, North America and tropical Africa. The nominate subspecies occurs in northern Europe (Ackery et al., 1995). Shields & Montgomery (1966) mentioned that European texts list Polygonaceae (Rumex and Polygonum) as larval foodplants for L. phlaeas subspecies. Flight period is April to November, in one to four generations, depending on local conditions; over-wintering is in the larval stage (Tuzov, 2000). Bridges (1988) listed 19 subspecies in his catalogue, not including the North American ones. Miller and Brown (1981) listed five subspecies for North America. Ford (1924) attempted to cover the world-wide geographic races of L. -
Description of a New Subspecies of Polyommatus Ciloicus De Freina & Witt, 1983: Alamuticus Ssp
Nachr. entomol. Ver. Apollo, N. F. 27 (3): 171–175 (2006) 171 Description of a new subspecies of Polyommatus ciloicus de Freina & Witt, 1983: alamuticus ssp. n. from North Iran (Alburz Mts.) (Lepidoptera: Lycaenidae) Alireza Naderi and Wolfgang ten Hagen Alireza Naderi, National Natural History Museum of Iran, Tehran, Iran; [email protected] Dr. Wolfgang ten Hagen, Frühlingstraße 1, D-63853 Mömlingen, Germany; [email protected] Abstract: In the present paper a new subspecies of Poly subspecies of P. ciloicus has been detected and described ommatus ciloicus de Freina & Witt, 1983 from western from NW Iran, province Azarbayjan-e Gharbi: P. ciloicus part of Central Alburz (Iran) is described and illustrated: azarisorum Weidenhoffer, 2002. alamuticus ssp. n. (male holotype in coll. Pest and Plant Diseases Research Institute [PPDRI], Tehran, Iran). Males We also provide interesting new distributional infor- of the new taxon can be distinguished by broader wings, mation about the presence of the scarce Pseudophilotes deep blue colour with completely black marginal line on bavius (Eversmann, 1832) at the type locality of the new upperside, fine and definitive markings of underside and totally white fringes. Information on ecology and distribu- subspecies. tion is provided. P. ciloicus from Qazvin can be distinguished from both other subspecies and is described as follows: Eine neue Unterart von Polyommatus ciloicus de Freina & Witt, 1983: alamuticus ssp. n. aus Nordiran Polyommatus ciloicus alamuticus ssp. n. (Lepidoptera: Lycaenidae) Holotype ♂: Iran, Qazvin prov., N. Qazvin, 2000 m, 23. v. Zusammenfassung: Eine neue Subspezies von Polyomma 2005, leg. A. R. Naderi, coll. Pest and Plant Diseases Research tus ciloicus de Freina & Witt, 1983 wird aus dem westlichen Institute (PPDRI), Tehran, Iran. -
Butterflies of the Balkans - Bulgaria, Macedonia and Greece Rd Th Sunday 3 - Sunday 17 July 2016 Trip Report by Dr
Butterflies of the Balkans - Bulgaria, Macedonia and Greece rd th Sunday 3 - Sunday 17 July 2016 Trip report by Dr. Mario Langourov Tour leader: Dr. Mario Langourov1 Tour participants: Mr. Kenneth Bailey Mr. Stephen Meredith Mr. Peter Chadd Mr. Adrian Hoskins Mr.Patrick Nash rd th th th th Bulgaria, 3 – 5 , 19 and 13 – 17 July 2016 th th Macedonia, 5 – 9 July th th Greece, 9 – 12 July rd Day 1 Sunday 3 July Bulgaria – Outbound from Sofia; en route to Rila Monastery. Weather: sunny with some clouds, still & warm (~23ºC). Before noon I welcomed three of the participants at the Terminal 1 and together we went to Terminal 2, where the others were. Before we head for our first hotel, located near the Rila Monastery, we stopped not far from Sofia. There, among the fresh greenery, we found mud puddling Lesser Purple Emperor (Pic. 2) and Purple Emperor, and on the surrounding bushes - Map Butterfly, Holly Blue, Speckled Wood. On the road landed and posed Great Banded Grayling. When we swerved off the main road, we decided to stop and that allowed us to expand the list of the day with Scarce Swallowtail Adonis Blue Purple-shot Copper. On the branches of the bushes we saw Sloe Hairstreak and Ilex Hairstreak, along the stream landed Zephyr Blue and Sandy Grizzled Skipper (Pic. 3). Lattice Brown looked for the deep shadows under the overhanging branches, while Large Blue and Checkered Blue prefered the proximity of its food plants. But we are low on time and we have no choice, but to set off to the hotel. -
Ripartii (Freyer, 1830) (Lepidoptera, Lycaenidae) in Croatia
NAT. CROAT. VOL. 19 No 2 46367 –4 ZAGREB December 31, 2010 short communication/kratko priop}enje FIRST FINDING OF RIPART’S ANOMALOUS BLUE POLYOMMATUS (AGRODIAETUS) RIPARTII (FREYER, 1830) (LEPIDOPTERA, LYCAENIDAE) IN CROATIA TONI KOREN Univerza na Primorskem, Znanstveno-raziskovalno sredi{~e Koper, In{titut za biodiverzitetne {tudije, Garibaldijeva 1, SI-6000 Koper, Slovenija ([email protected]) Koren, T.: First finding of Ripart’s Anomalous Blue Polyommatus (Agrodiaetus) ripartii (Freyer, 1830) (Lepidoptera, Lycaenidae) in Croatia. Nat. Croat., Vol. 19, No. 2., 463–467, 2010, Zagreb. The first record of Ripart’s Anomalous Blue Polyommatus (Agrodiaetus) ripartii (Freyer, 1830) (Lepidoptera, Lycaenidae) in Croatia is presented. A single specimen was found during field re- search in August 2010 between the village of Zrmanja and Zrmanja spring (300 m a.s.l.). This re- cord at least partially fills the gap in the distribution of P. ripartii in Europe and indicates a possibil- ity that more unrecorded species will be found in Croatia. Ripart’s Anomalous Blue is the 194th butterfly species recorded in Croatia. Key words: Polyommatus (Agrodiaetus) ripartii, faunistics, butterflies, Zrmanja, first record Koren, T.: Prvi nalaz Ripartijevog sme|eg plavca Polyommatus (Agrodiaetus) ripartii (Freyer, 1830) (Lepidoptera, Lycaenidae) u Hrvatskoj. Nat. Croat., Vol. 19, No. 2., 463–467, 2010, Zagreb. U ovom radu opisuje se prvi nalaz Ripartijevog sme|eg plavca Polyommatus (Agrodiaetus) ripartii (Freyer, 1830) u Hrvatskoj. Vrsta je prona|ena tijekom terenskih istra`ivanja danjih leptira u kolo- vozu 2010. godine na 300 metara nadmorske visine izme|u sela Zrmanja i izvora rijeke Zrmanje. Ovaj nalaz donekle upotpunjuje prazninu u distribuciji ove vrste u Europi te otvara mogu}nost pronalaska novih vrsta danjih leptira u Hrvatskoj. -
The Recent Diversification of the Lysandra Butterflies Through Chromosomal Change
SUPPLEMENTARY MATERIAL In the shadow of phylogenetic uncertainty: the recent diversification of the Lysandra butterflies through chromosomal change Gerard Talaveraa,b,c, Vladimir A. Lukhtanovb,d, Lukas Rieppelc,e, Naomi E. Piercec and Roger Vilaa,* aInstitut de Biologia Evolutiva (CSIC-UPF), Passeig Marítim de la Barceloneta, 37, 08003 Barcelona, Spain b Faculty of Biology & Soil Science, St Petersburg State University, Universitetskaya nab. 7/9, 199034 St Petersburg, Russia c Department of Organismic and Evolutionary Biology and Museum of Comparative Zoology, Harvard University, 26 Oxford Street, Cambridge, Massachusetts 02138, USA d Department of Karyosystematics, Zoological Institute of Russian Academy of Science, Universitetskaya nab. 1, 199034 St Petersburg, Russia e Department of History, Brown University, 79 Brown Street, Providence, RI 02192, USA *Corresponding author: [email protected] SUPPLEMENTARY DISCUSSION Phylogenetic relationships We recover three well-differentiated clades plus three species with apparently no close relative (L. syriaca, L. dezina and L. ossmar). One of the strongly supported clades is formed by L. punctifera and L. bellargus, a grouping that corresponds very well to morphology. In fact L. punctifera and L. bellargus are so similar in their wing patterns that the taxon punctifera, first described by Oberthür in 1876 was initially considered a subspecies of L. bellargus. Much later, de Lesse (1959) assigned punctifera species status based on differences in chromosome number. These two taxa split ca. 0.74 Mya, possibly because of dispersal across the West Mediterranean (perhaps through the Gibraltar Strait), since L. bellargus is widespread in the Iberian Peninsula and across Europe into Western Asia, while L. punctifera is confined to the Southwestern Mediterranean shore (Morocco, N. -
Recent Literature on Lepidoptera
1957 The Lepidopterists' News 233 RECENT LITERATURE ON LEPIDOPTERA (U nder the supervision of PETER F. BELLINGER) B. SYSTEMATICS AND NOMENCLATURE d'Almeida, R Ferreira, "Ligeiras notas sabre Ithomiidreda America do Sui (Lep., Ithomiidre)" [in Spanish]. An. Inst. BioI., Mexico, vol. 20: pp. 393-397, 3 figs. 1949. Describes as new Mechanitis elisa acrreana (Xapuri, Territorio do Acre, Brazil); Dirrell1za acreana (Xapuri); Sinks 11 thesis clearista bassleri to A. Co columbiensis Kaye; lists new entities described by Kaye in paper (1918 ) omitted from Lepidop !e;-orulll Cata/oglls. Note on Hypothyris ben ella. [Po B.J d' Almeida, R Ferriera, "Ligeiras observa~oes sabre 0 genero Cithrerias Hiibner, 1819. (Lep. Satyridre)" [in Portuguese]. Arg. Zool. Est. Slio Paulo, vol. 7: pp. 493-505, 2 pis., 1 fig. 11 June 1951. Describes as new C. similigena (Sao Joaquim, R. I<;ana, Amazonas, Brazil); C. jllruaiillsis (Porto Walter, Alto Rio Jurua, Acre. Brazil); DULCEDO (type Hertera polita). Briefly redescribes C. pireta, C. phantoma, & C. aurorilla ,. discllsses taxollornic histury of genus; gives a tentative catalogue; records distribution of spp. known to author. [Po B.J d'Almeida, R. Ferreira, " Notas sinonfmicas s6bre Ithomiidre (Lepidoptera, Rhopalo cera)" [in Portuguese]. Bol. Mus. Nar., Rio de Janeiro, Zoo I., no. 143: 18 pp., 21 figs. 24 Sept. 19 56 . Describes as new Ceratinia nise zikani (Utinga, Beh'm, Para, Brazil). Notes on spp. & sspp. described by Zikan, 1940-42, & by Bryk, 1953, with some new synonymy; figures some adults, & c; genitalia of Hypothyris antonina. [Po B.J Eliot, N ., "The strange case of the Camberwell Beauty." Entomologist, vol. -
Price/Contents
European Butterflies: A Portrait in Photographs CONTENTS and PRICES Papilionidae, Pieridae, Lycaenidae, Riodinidae, Nymphalidae (part) The species in each chapter and their subspecies (if more than one) are listed below (forms are not included). Certain taxa, treated as species, subspecies or forms else- where, may be ranked differently in the present publication. The list includes: chapter number and title; number of printed pages (excluding contents page / cover); price of loose-leaf version (includes contents page); price of bound version (includes covers / contents page); and price of digital (pdf) version per batch. 3.0. Papilionidae Introduction 8pp £2.00 / 2.70 3.1. Swallowtail Group 29pp £6.20 / 6.90 Papilio machaon (Swallowtail) P. m. machaon, P. m. britannicus Papilio hospiton (Corsican Swallowtail) Papilio alexanor (Southern Swallowtail) Iphiclides podalirius (Scarce Swallowtail) I. p. podalirius, I. p. feisthamelii 3.2. Festoon Group 27pp £5.80 / 6.50 Zerynthia cerisyi (Eastern Festoon) Zerynthia cretica (Cretan Festoon) Zerynthia polyxena (Southern Festoon) Z. p. polyxena, Z. p. cassandra Zerynthia rumina (Spanish Festoon) Archon apollinus (False Apollo) 3.3. Apollo Group 25pp £5.40 / 6.10 Parnassius apollo (Apollo) P. a. apollo, P. a. nevadensis, P. a. pumilus Parnassius phoebus (Small Apollo) Parnassius mnemosyne (Clouded Apollo) Batch of chs 3.0 to 3.3 89pp £19.40 (loose-leaf) £22.20 (booklets) £4.85 (digital) page 1 copyright © B R Watts, October 2017 European Butterflies: A Portrait in Photographs 4.0. Pieridae Introduction 8pp £2.00 / 2.70 4.1. Large and Small White Group 57pp £11.80 / 12.50 Aporia crataegi (Black-veined White) Pieris brassicae (Large White) Pieris rapae (Small White) Pieris mannii (Southern Small White) Pieris ergane (Mountain Small White) 4.2. -
Lepidoptera: Lycaenidae)
ZOBODAT - www.zobodat.at Zoologisch-Botanische Datenbank/Zoological-Botanical Database Digitale Literatur/Digital Literature Zeitschrift/Journal: Nachrichten des Entomologischen Vereins Apollo Supplement Jahr/Year: 1997 Band/Volume: 16 Autor(en)/Author(s): Eckweiler Wolfgang, Häuser Christoph L. Artikel/Article: An illustrated checklist of Agrodiaetus Hübner, 1822, a subgenus of Polyommatus Latreille, 1804 113-168 ©Entomologischer Verein Apollo e.V. Frankfurt am Main; download unter www.zobodat.at Nachr. entomol. Ver. Apollo, Suppl. 16: 113-168 (1997) 113 An illustrated checklist of Agrodiaetus Hübner, 1822, a subgenus of Polyommatus Latreille, 1804 (Lepidoptera: Lycaenidae) Wolfgang E ck We il e r and Christoph L. H ä u ser Dr. Wolfgang E ckweiler, Gronauer Strasse 40, D-60385 Frankfurt am Main Dr. Christoph L. Häuser, Staatliches Museum für Naturkunde, Rosenstein 1, D-70191 Stuttgart Abstract: This checklist of the subgenus Agrodiaetus H übner, 1822 of Polyom matus Latreille, 1804 contains 190 available species-group names, which are presented in a tentative systematic arrangement and also in alphabetic form. Chromosome numbers known are stated for individual taxa, and nearly all recognised species are illustrated in colour. Systematisches Verzeichnis von Agrodiaetus Hübner , 1822, einer Unter gattung von Polyommatus Latreille, 1804 (Lepidoptera: Lycaenidae) Zusammenfassung: In dieser Arbeit werden 190 verfügbare und gültige Taxa der zu Polyommatus Latreille, 1804 gehörenden Untergattung Agrodiaetus H übner, 1822 systematisch und auch alphabetisch aufgelistet. Die Chromoso menzahl der einzelnen Taxa werden — soweit bekannt — angegeben und fast alle Agrodiaetus-Arten werden farbig abgebildet. Introduction The subgenus Agrodiaetus H ü b n e r comprises to date 190 available spe cies-group taxa (H ä u ser & E c k w eiler 1997), more than any other group included in the genus Polyommatus L a t r e il l e , 1804. -
Phylogeny of European Butterflies V1.0
bioRxiv preprint doi: https://doi.org/10.1101/844175; this version posted November 16, 2019. The copyright holder for this preprint (which was not certified by peer review) is the author/funder, who has granted bioRxiv a license to display the preprint in perpetuity. It is made available under aCC-BY 4.0 International license. A complete time-calibrated multi-gene phylogeny of the European butterflies Martin Wiemers1,2*, Nicolas Chazot3,4,5, Christopher W. Wheat6, Oliver Schweiger2, Niklas Wahlberg3 1Senckenberg Deutsches Entomologisches Institut, Eberswalder Straße 90, 15374 Müncheberg, Germany 2UFZ – Helmholtz Centre for Environmental Research, Department of Community Ecology, Theodor- Lieser-Str. 4, 06120 Halle, Germany 3Department of Biology, Lund University, 22362 Lund, Sweden 4Department of Biological and Environmental Sciences, University of Gothenburg, Box 461, 405 30 Gothenburg, Sweden. 5Gothenburg Global Biodiversity Centre, Box 461, 405 30 Gothenburg, Sweden. 6Department of Zoology, Stockholm University, 10691 Stockholm, Sweden *corresponding author: e-mail: [email protected] Abstract With the aim of supporting ecological analyses in butterflies, the third most species-rich superfamily of Lepidoptera, this paper presents the first time-calibrated phylogeny of all 496 extant butterfly species in Europe, including 18 very localized endemics for which no public DNA sequences had been available previously. It is based on a concatenated alignment of the mitochondrial gene COI and up to 11 nuclear gene fragments, using Bayesian inference of phylogeny. To avoid analytical biases that could result from our region-focus sampling, our European tree was grafted upon a global genus- level backbone butterfly phylogeny for analyses. In addition to a consensus tree, we provide the posterior distribution of trees and the fully-concatenated alignment for future analyses.