Environmental Assessment of Battery Systems: Critical Issues for Established and Emerging Technologies
Total Page:16
File Type:pdf, Size:1020Kb
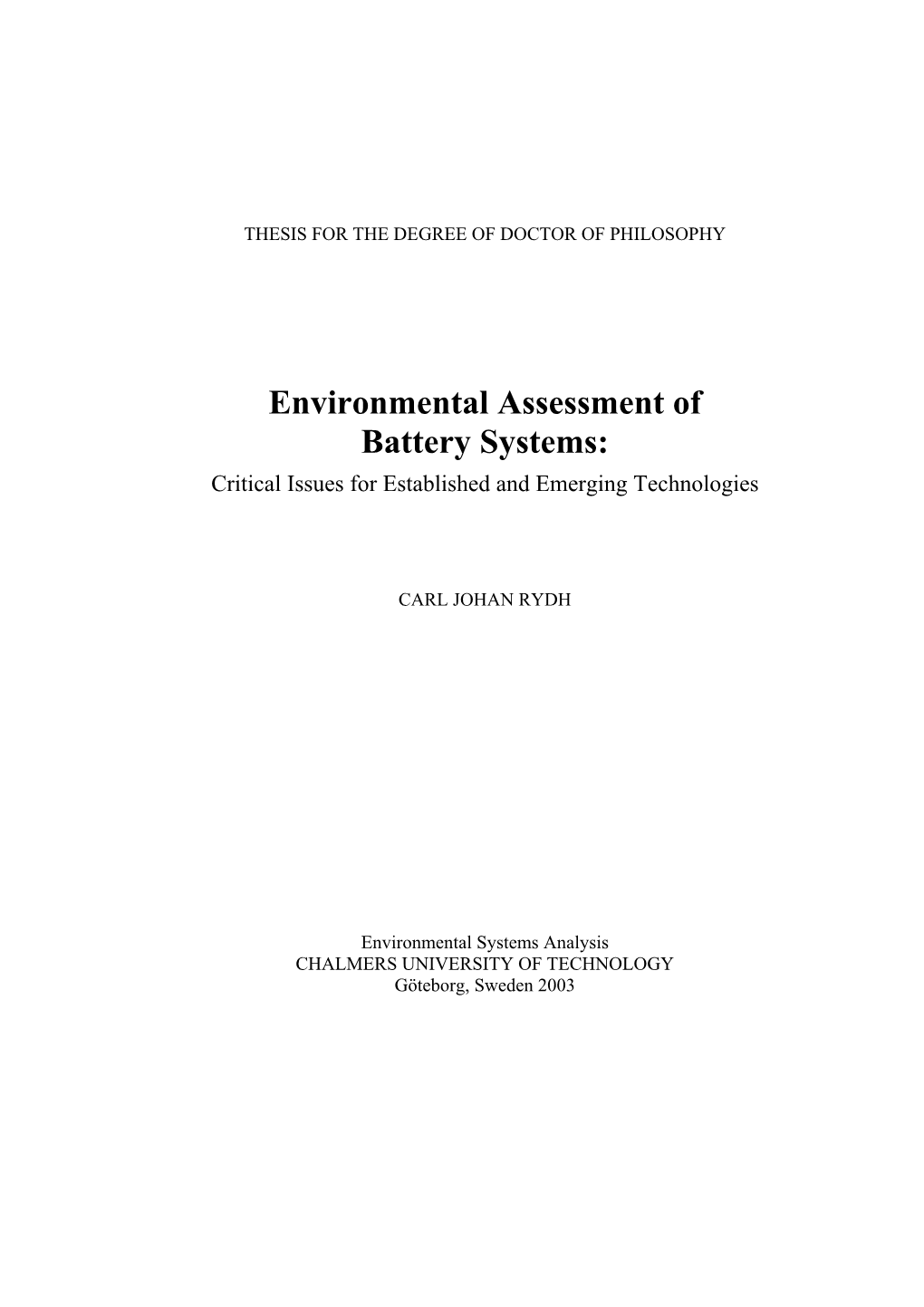
Load more
Recommended publications
-
In-Situ Tools Used in Vanadium Redox Flow Battery Research—Review
batteries Review In-Situ Tools Used in Vanadium Redox Flow Battery Research—Review Purna C. Ghimire 1,*, Arjun Bhattarai 1,*, Tuti M. Lim 2, Nyunt Wai 3 , Maria Skyllas-Kazacos 4 and Qingyu Yan 5,* 1 V-flow Tech Pte Ltd., Singapore, 1 Cleantech Loop, Singapore 637141, Singapore 2 School of Civil and Environmental Engineering, Nanyang Technological University, 50 Nanyang Avenue, Singapore 639798, Singapore; [email protected] 3 Energy Research Institute @Nanyang Technological University, 1 Cleantech Loop, Singapore 637141, Singapore; [email protected] 4 School of Chemical Engineering, The University of New South Wales, Sydney 2052, Australia; [email protected] 5 School of Material Science and Engineering, Nanyang Technological University, Singapore 637141, Singapore * Correspondence: purna.ghimire@vflowtech.com (P.C.G.); arjun.bhattarai@vflowtech.com (A.B.); [email protected] (Q.Y.); Tel.: +65-85153215 (P.C.G.) Abstract: Progress in renewable energy production has directed interest in advanced developments of energy storage systems. The all-vanadium redox flow battery (VRFB) is one of the attractive technologies for large scale energy storage due to its design versatility and scalability, longevity, good round-trip efficiencies, stable capacity and safety. Despite these advantages, the deployment of the vanadium battery has been limited due to vanadium and cell material costs, as well as supply issues. Improving stack power density can lower the cost per kW power output and therefore, intensive research and development is currently ongoing to improve cell performance by increasing electrode activity, reducing cell resistance, improving membrane selectivity and ionic conductivity, etc. In order Citation: Ghimire, P.C.; Bhattarai, A.; to evaluate the cell performance arising from this intensive R&D, numerous physical, electrochemical Lim, T.M.; Wai, N.; Skyllas-Kazacos, and chemical techniques are employed, which are mostly carried out ex situ, particularly on cell M.; Yan, Q. -
Characterization of Battery for Energy Storage Applications – Lead Acid
Abstract #1672, 224th ECS Meeting, © 2013 The Electrochemical Society Characterization of battery for energy storage applications curves of a lead acid battery (YUASA, NP4-6). It has a – lead acid battery, lithium battery, vanadium redox normal voltage of 6 V and capacity of 4 Ah. The battery flow battery, and capacitor power was drained at 0.5 A till the cell voltage down to 3.0 V. The E-I curve was recorded by linear scanning Chih-Lung Hsieh1, Yen-Ting Liu2, Kan-Lin Hsueh2, amperometry at scanning rate of 0.01 A/s. The battery is Ju-Shei Hung3 then charge at 0.5 A for 2 hours. The SOC was assumed reached 25%. The E-I curve was then recorded. This 1. Institute of Nuclear Energy Research, Taoyuan, Taiwan procedure was carried out for SOC at 50%, 75%, and 2. Dept. Energy Eng., National United University, Miaoli, 100%. When cell voltage reached 7.0 V during charge Taiwan was considered as 100% SOC. Curves shown on Fig. 2 3. Dept. Chem. Eng., National United University, Miaoli, was measured result of laed acid battery. These curves Taiwan can be described by following equations. Where the IR accounts for internal resistance. Last term of equation [1] is the voltage cahge due to acitvation over-potential. The purpose of this study is to measure the charge/discharge characteristics of four different During Charge components for energy storage application. They are lead RT nF E E 1 iRI o ln o exp[ )]( [1] acid battery, lithium battery, vanadium redox flow battery, nF SO 2 H 4 RT [ 4 [] ] and capacitor. -
Current State and Future Prospects for Electrochemical Energy Storage and Conversion Systems
energies Review Current State and Future Prospects for Electrochemical Energy Storage and Conversion Systems Qaisar Abbas 1 , Mojtaba Mirzaeian 2,3,*, Michael R.C. Hunt 1, Peter Hall 2 and Rizwan Raza 4 1 Centre for Materials Physics, Department of Physics, Durham University, Durham DH1 3LE, UK; [email protected] (Q.A.); [email protected] (M.R.H.) 2 School of Computing, Engineering and Physical Sciences, University of the West of Scotland, Paisley PA1 2BE, UK; [email protected] 3 Faculty of Chemistry and Chemical Technology, Al-Farabi Kazakh National University, Al-Farabi Avenue, 71, Almaty 050040, Kazakhstan 4 Clean Energy Research Lab (CERL), Department of Physics, COMSATS University Islamabad, Lahore 54000, Pakistan; [email protected] * Correspondence: [email protected] Received: 30 September 2020; Accepted: 26 October 2020; Published: 9 November 2020 Abstract: Electrochemical energy storage and conversion systems such as electrochemical capacitors, batteries and fuel cells are considered as the most important technologies proposing environmentally friendly and sustainable solutions to address rapidly growing global energy demands and environmental concerns. Their commercial applications individually or in combination of two or more devices are based on their distinguishing properties e.g., energy/power densities, cyclability and efficiencies. In this review article, we have discussed some of the major electrochemical energy storage and conversion systems and encapsulated their technological advancement in recent years. Fundamental working principles and material compositions of various components such as electrodes and electrolytes have also been discussed. Furthermore, future challenges and perspectives for the applications of these technologies are discussed. -
Recent Progress in Vanadium Redox-Flow Battery Katsuji Emura1 Sumitomo Electric Industries, Ltd., Osaka, Japan
Recent Progress in Vanadium Redox-Flow Battery Katsuji Emura1 Sumitomo Electric Industries, Ltd., Osaka, Japan 1. Introduction Vanadium Redox Flow Battery (VRB) is an energy storage system that employs a rechargeable vanadium fuel cell technology. Since 1985, Sumitomo Electric Industries Ltd (SEI) has developed VRB technologies for large-scale energy storage in collaboration with Kansai Electric power Co. In 2001, SEI has developed 3MW VRB system and delivered it to a large liquid crystal display manufacturing plant. The VRB system provides 3MW of power for 1.5 seconds as UPS (Uninterruptible Power Supply) for voltage sag compensation and 1.5MW of power for 1hr as a peak shaver to reduce peak load. The VRB system has successfully compensated for 50 voltage sag events that have occurred since installation up until September 2003. 2. Principle of VRB The unique chemistry of vanadium allows it to be used in both the positive and negative electrolytes. Figure 1 shows a schematic configuration of VRB system. The liquid electrolytes are circulated through the fuel cells in a similar manner to that of hydrogen and oxygen in a hydrogen fuel cell. Similarly, the electrochemical reactions occurring within the cells can produce a current flow in an external circuit, alternatively reversing the current flow results in recharging of the electrolytes. These cell reactions are balanced by a flow of protons or hydrogen ions across the cell through a selective membrane. The selective membrane also serves as a physical barrier keeping the positive and negative vanadium electrolytes separate. Figure 2 shows a cell stack which was manufactured by SEI. -
Battery Technologies for Small Scale Embeded Generation
Battery Technologies for Small Scale Embedded Generation. by Norman Jackson, South African Energy Storage Association (SAESA) Content Provider – Wikipedia et al Small Scale Embedded Generation - SSEG • SSEG is very much a local South African term for Distributed Generation under 10 Mega Watt. Internationally they refer to: Distributed generation, also distributed energy, on-site generation (OSG) or district/decentralized energy It is electrical generation and storage performed by a variety of small, grid- connected devices referred to as distributed energy resources (DER) Types of Energy storage: • Fossil fuel storage • Thermal • Electrochemical • Mechanical • Brick storage heater • Compressed air energy storage • Cryogenic energy storage (Battery Energy • Fireless locomotive • Liquid nitrogen engine Storage System, • Flywheel energy storage • Eutectic system BESS) • Gravitational potential energy • Ice storage air conditioning • Hydraulic accumulator • Molten salt storage • Flow battery • Pumped-storage • Phase-change material • Rechargeable hydroelectricity • Seasonal thermal energy battery • Electrical, electromagnetic storage • Capacitor • Solar pond • UltraBattery • Supercapacitor • Steam accumulator • Superconducting magnetic • Thermal energy energy storage (SMES, also storage (general) superconducting storage coil) • Chemical • Biological • Biofuels • Glycogen • Hydrated salts • Starch • Hydrogen storage • Hydrogen peroxide • Power to gas • Vanadium pentoxide History of the battery This was a stack of copper and zinc Italian plates, -
2020 Grid Energy Storage Technology Cost and Performance Assessment
Energy Storage Grand Challenge Cost and Performance Assessment 2020 December 2020 2020 Grid Energy Storage Technology Cost and Performance Assessment Kendall Mongird, Vilayanur Viswanathan, Jan Alam, Charlie Vartanian, Vincent Sprenkle*, Pacific Northwest National LaBoratory. Richard Baxter, Mustang Prairie Energy * [email protected] Technical Report Publication No. DOE/PA-0204 December 2020 Energy Storage Grand Challenge Cost and Performance Assessment 2020 December 2020 Disclaimer This report was prepared as an account of work sponsored by an agency of the United States government. Neither the United States government nor any agency thereof, nor any of their employees, makes any warranty, express or implied, or assumes any legal liability or responsibility for the accuracy, completeness, or usefulness of any information, apparatus, product, or process disclosed, or represents that its use would not infringe privately owned rights. Reference herein to any specific commercial product, process, or service by trade name, trademark, manufacturer, or otherwise does not necessarily constitute or imply its endorsement, recommendation, or favoring by the United States government. ii Energy Storage Grand Challenge Cost and Performance Assessment 2020 December 2020 Acronyms AC alternating current Ah ampere-hour BESS battery energy storage system BLS U.S. Bureau of Labor Statistics BMS battery management system BOP balance of plant BOS balance of system C&C controls & communication C&I civil and infrastructure CAES compressed-air energy -
Advanced Battery Storage Systems Testing at ACEP
Advanced Battery Storage Systems Testing at ACEP THE PRUDENT ENERGY VRB-ESS Characterization and Assessment of the Flow Battery Concept for Energy Storage and Ancillary Services in Isolated Wind-Diesel Power Networks in Alaska March 2012 Cover photo: The power conversion system of the 5 kw, 20 kWh Vanadium Redox Flow Battery courtesy of Prudent Energy. Any comments or suggestions may be directed to the authors at the respective addresses below. Billy Muhando Asst Research Prof Alaska Center for Energy and Power (ACEP) University of Alaska Fairbanks [email protected] 1 907 474 7082 Tom Johnson Research Engineer Alaska Center for Energy and Power (ACEP) University of Alaska Fairbanks [email protected] 1 907 474 5564 Further information may be obtained by contacting: Gwen Holdmann Director Alaska Center for Energy and Power (ACEP) University of Alaska [email protected] 1 907 474 5402 www.uaf.edu/acep Disclaimer This report was prepared by the Alaska Center for Energy and Power (ACEP) as an account of work sponsored by an agency of the United States Government – the Denali Commission – and is disseminated in the interest of information exchange and general guidance only. Neither the United States Government nor any agency thereof, nor the state of Alaska, nor any of their employees, makes any warranty, express or implied, or assumes any legal liability or responsibility for the accuracy, completeness, or usefulness of any information, apparatus, product, or process disclosed, or represents that its use would not infringe privately owned rights. Reference herein to any specific commercial product, process, or service by trade name, trademark, manufacturer, or otherwise does not necessarily constitute or imply its endorsement, recommendation, or favoring by the United States Government, the state of Alaska, or any agency thereof. -
New Flow Battery to Keep Big Cities Lit, Green and Safe
New flow battery to keep big cities lit, green and safe Wed, 02/25/2015 - 10:27am Frances White, PNNL Get today's R&D headlines and news - Sign up now! Ensuring the power grid keeps the lights on in large cities could be easier with a new battery design that packs far more energy than any other battery of its kind and size. The new zinc-polyiodide redox flow battery, described in Nature Communications , uses an electrolyte that has more than two times the energy density of the next-best flow battery used to store renewable energy and support the power grid. And its energy density is approaching that of a type of lithium-ion battery used to power portable electronic devices and some small electric vehicles. "With improved energy density and inherent fire safety, flow batteries could provide long- duration energy storage for the tight confines of urban settings, where space is at a premium," said Imre Gyuk, energy storage program manager at the U.S. Dept. of Energy (DOE)'s Office of Electricity Delivery and Energy Reliability, which funded this research. "This would enhance the resiliency and flexibility of the local electrical grid." "Another, unexpected bonus of this electrolyte's high energy density is it could potentially expand the use of flow batteries into mobile applications such as powering trains and cars," said the study's corresponding author, Wei Wang, a materials scientist at DOE's Pacific Northwest National Laboratory (PNNL). Going with the flow Both flow and lithium-ion batteries were invented in the 1970s, but only the lithium-ion variety took off at that time. -
Enhanced Cycling Performance of Rechargeable Zinc–Air Flow Batteries Using Potassium Persulfate As Electrolyte Additive
International Journal of Molecular Sciences Article Enhanced Cycling Performance of Rechargeable Zinc–Air Flow Batteries Using Potassium Persulfate as Electrolyte Additive Ramin Khezri 1 , Soraya Hosseini 1, Abhishek Lahiri 2, Shiva Rezaei Motlagh 3, Mai Thanh Nguyen 4 , Tetsu Yonezawa 4,5 and Soorathep Kheawhom 1,6,* 1 Department of Chemical Engineering, Faculty of Engineering, Chulalongkorn University, Bangkok 10330, Thailand; [email protected] (R.K.); [email protected] (S.H.) 2 Department of Chemical Engineering, Brunel University London, London UB8 3PH, UK; [email protected] 3 Department of Chemical Engineering, Faculty of Engineering, Universiti Putra Malaysia, Selangor 43300, Malaysia; [email protected] 4 Division of Materials Science and Engineering, Faculty of Engineering, Hokkaido University, Hokkaido 060-8628, Japan; [email protected] (M.T.N.); [email protected] (T.Y.) 5 Institute for the Promotion of Business-Regional Collaboration, Hokkaido University, Sapporo 001-0021, Japan 6 Research Unit of Advanced Materials for Energy Storage, Chulalongkorn University, Bangkok 10330, Thailand * Correspondence: [email protected] Received: 3 September 2020; Accepted: 1 October 2020; Published: 2 October 2020 Abstract: Zinc–air batteries (ZABs) offer high specific energy and low-cost production. However, rechargeable ZABs suffer from a limited cycle life. This paper reports that potassium persulfate (KPS) additive in an alkaline electrolyte can effectively enhance the performance and electrochemical characteristics of rechargeable zinc–air flow batteries (ZAFBs). Introducing redox additives into electrolytes is an effective approach to promote battery performance. With the addition of 450 ppm KPS, remarkable improvement in anodic currents corresponding to zinc (Zn) dissolution and limited passivation of the Zn surface is observed, thus indicating its strong effect on the redox reaction of Zn. -
High Current Density Redox Flow Batteries for Stationary Electrical Energy Storage
PNNL REPORT PNNL-23819-4 Unlimited Release High Current Density Redox Flow Batteries for Stationary Electrical Energy Storage Milestone Report for the DOE-OE Energy Storage Systems Program (FY16 Quarter 4: October 2015 through September 2016) David Reed, Ed Thomsen, Vilayanur Viswanathan, Wei Wang, Zimin Nie and Vincent Sprenkle Prepared by Pacific Northwest National Laboratory Richland, Washington 99354 Pacific Northwest National Laboratory is a multi-program laboratory managed and operated by Battelle Memorial Institute for the U.S. Department of Energy under contract DE-AC05-76RL01830. Approved for public release; further dissemination unlimited. Issued by Pacific Northwest National Laboratory, operated for the United States Department of Energy by Battelle Memorial Institute NOTICE: This report was prepared as an account of work sponsored by an agency of the United States Government. Neither the United States Government, nor any agency thereof, nor any of their employees, nor any of their contractors, subcontractors, or their employees, make any warranty, express or implied, or assume any legal liability or responsibility for the accuracy, completeness, or usefulness of any information, apparatus, product, or process disclosed, or represent that its use would not infringe privately owned rights. Reference herein to any specific commercial product, process, or service by trade name, trademark, manufacturer, or otherwise, does not necessarily constitute or imply its endorsement, recommendation, or favoring by the United States Government, any agency thereof, or any of their contractors or subcontractors. The views and opinions expressed herein do not necessarily state or reflect those of the United States Government, any agency thereof, or any of their contractors. -
Battery Storage Technology, Systems and Cost Trends
Energy Storage Technology Advancement Partnership (ESTAP) Webinar Energy Storage 101, Part 1: Battery Storage Technology, Systems and Cost Trends March 26, 2019 Housekeeping Join audio: • Choose Mic & Speakers to use VoIP • Choose Telephone and dial using the information provided Use the orange arrow to open and close your control panel Submit questions and comments via the Questions panel This webinar is being recorded. We will email you a webinar recording within 48 hours. This webinar will be posted on CESA’s website at www.cesa.org/webinars www.cesa.org Energy Storage Technology Advancement Partnership (ESTAP) (bit.ly/ESTAP) ESTAP is supported by the U.S. Department of Energy Office of Electricity and Sandia National Laboratories, and is managed by CESA. ESTAP Key Activities: ESTAP Project Locations: New Jersey: $10 1. Disseminate information to stakeholders New York: $40 Vermont: 4 MW Oregon: 500 kW million, 4-year Massachusetts: $40 Million Million energy storage Energy Storage energy storage Resilient Power/Microgrids Microgrids microgrid & Demonstration solicitation: 13 Solicitation: 11 projects • ESTAP listserv >5,000 members Initiative Airport Microgrid Project projects $10 Million energy storage • Webinars, conferences, information demo program updates, surveys. Connecticut: $50 Million, New Mexico: 3-year Microgrids Energy Storage 2. Facilitate public/private partnerships to support joint Initiative: 11 projects Task Force federal/state energy storage demonstration project deployment Pennsylvania Alaska: Kodiak Battery 3. Support state energy storage efforts with technical, policy Island Demonstration Wind/Hydro/ Project and program assistance Battery & Cordova hydro/battery Northeastern Maryland Game Changer Awards: projects States Post-Sandy Solar/EV/Battery Critical & Resiliency Through Microgrids Infrastructure Task Force Hawaii: 6MW Resiliency Project storage on Molokai Island and HECO projects 4 Webinar Speakers Dr. -
Vanadium Redox Flow Batteries: a Review Oriented to Fluid-Dynamic Optimization
energies Review Vanadium Redox Flow Batteries: A Review Oriented to Fluid-Dynamic Optimization Iñigo Aramendia 1,* , Unai Fernandez-Gamiz 1 , Adrian Martinez-San-Vicente 1, Ekaitz Zulueta 2 and Jose Manuel Lopez-Guede 2 1 Nuclear Engineering and Fluid Mechanics Department, University of the Basque Country UPV/EHU, Nieves Cano 12, 01006 Vitoria-Gasteiz, Spain; [email protected] (U.F.-G.); [email protected] (A.M.-S.-V.) 2 Automatic Control and System Engineering Department, University of the Basque Country UPV/EHU, Nieves Cano 12, 01006 Vitoria-Gasteiz, Spain; [email protected] (E.Z.); [email protected] (J.M.L.-G.) * Correspondence: [email protected]; Tel.: +34-945-014-066 Abstract: Large-scale energy storage systems (ESS) are nowadays growing in popularity due to the increase in the energy production by renewable energy sources, which in general have a random intermittent nature. Currently, several redox flow batteries have been presented as an alternative of the classical ESS; the scalability, design flexibility and long life cycle of the vanadium redox flow battery (VRFB) have made it to stand out. In a VRFB cell, which consists of two electrodes and an ion exchange membrane, the electrolyte flows through the electrodes where the electrochemical reactions take place. Computational Fluid Dynamics (CFD) simulations are a very powerful tool to develop feasible numerical models to enhance the performance and lifetime of VRFBs. This review aims to present and discuss the numerical models developed in this field and, particularly, to analyze different types of flow fields and patterns that can be found in the literature.