Ernest 0. Radiation Lawrence Laboratory
Total Page:16
File Type:pdf, Size:1020Kb
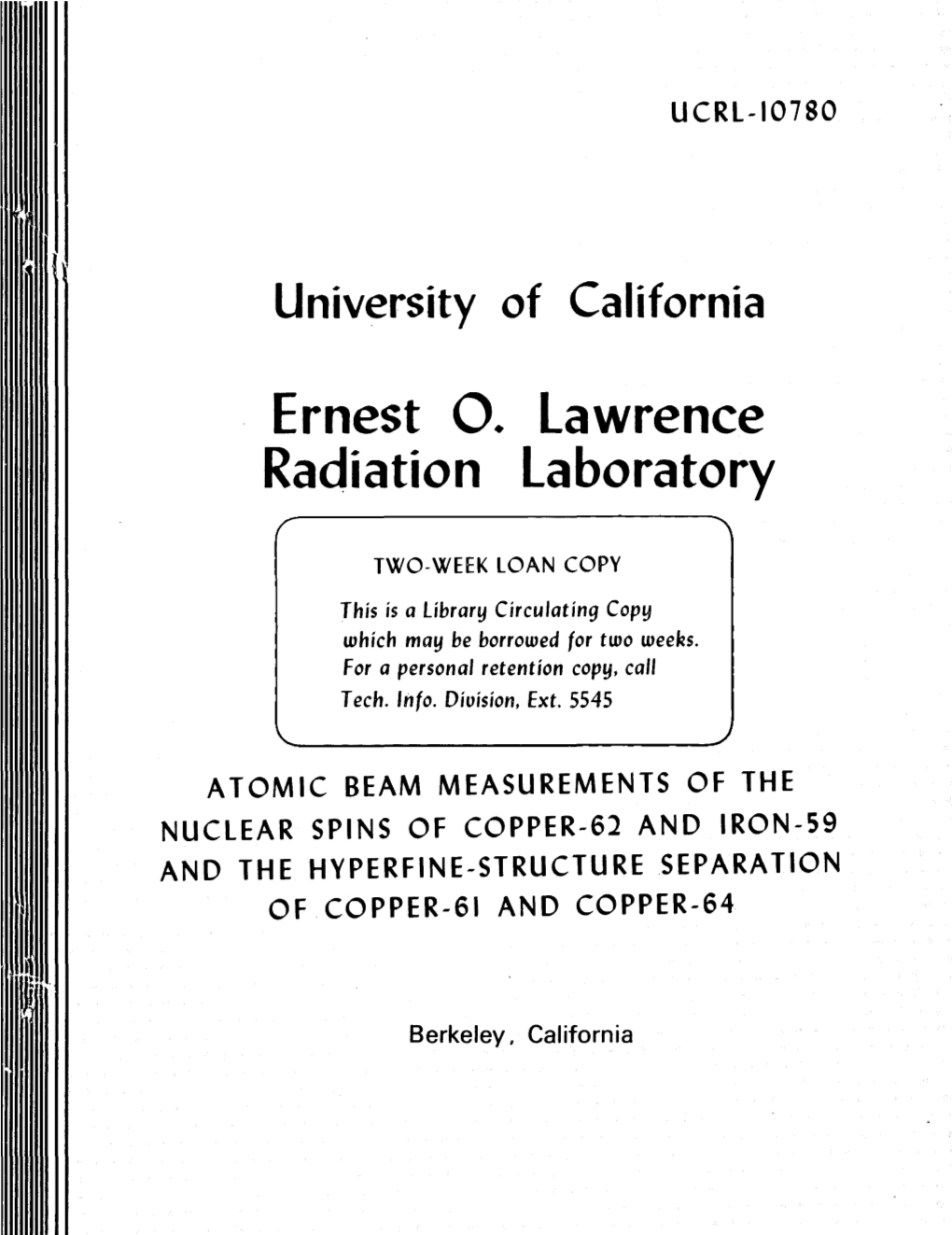
Load more
Recommended publications
-
Those Two Isotopes Are Chemical Identical and Can Only Be Separated by Using Their Relatively Small Difference in Mass
Exercise2. Why is it extremely difficult to separate the two isotopes of copper, 63Cu and 65Cu? Those two isotopes are chemical identical and can only be separated by using their relatively small difference in mass. Exercise 3. If a nuclear reaction adds an extra neutron to the nucleus of 57Fe (a stable isotope of iron), it produces 58Fe (another stable isotope of iron). Will this change in the nucleus affect the number and arrangement of the electrons in the atom that’s built around this nucleus? Why or why not? No: the number of electrons = number of protons (= atomic number) and this hasn’t changed. Because the number of electrons doesn’t change and the charge in the nucleus doesn’t change, the arrangement of electron orbitals (standing waves) also doesn’t change. Exercise 4: If a nuclear reaction adds an extra proton to the nucleus of 58Fe (a stable isotope of iron), it produces 59Co (a stable isotope of cobalt). Will this change in the nucleus affect the number and arrangement of the electrons in the atom that’s built around this nucleus? Why or why not? Yes: since the nuclear charge has increased, an additional electron is needed to form a neutral atom. Because of both the change in number of electrons and the stronger Coulomb attraction from the nucleus, the arrangement of electron orbitals will change. Exercise 7. When a large nucleus is split in half during an experiment at a nuclear physics lab, the result is usually two medium-size nuclei with too many neutrons to be stable. -
Cross Calibration for Using Neutron Activation Analysis with Copper Samples to Measure D-T Fusion Yields
Cross Calibration for using Neutron Activation Analysis with Copper Samples to measure D-T Fusion Yields Chad A. McCoy May 5, 2011 The University of New Mexico Department of Physics and Astronomy Undergraduate Honors Thesis Advisor: Gary W. Cooper (UNM ChNE) Daniel Finley Abstract We used a dense plasma focus with maximum neutron yield greater than 1012 neutrons per pulse as a D-T neutron source to irradiate samples of copper, praseodymium, silver, and lead, to cross- calibrate the coincidence system for using neutron activation analysis to measure total neutron yields. In doing so, we counted the lead samples using an attached plastic scintillator, due to the short half-life and single gamma decay. The copper samples were counted using two 6” NaI coincidence systems and a 3” NaI coincidence system to determine the total neutron yield. For the copper samples, we used a calibration method which we refer to as the “F factor” to calibrate the system as a whole and used this factor to determine the total neutron yield. We concluded that the most accurate measurement of the D-T fusion neutron yield using copper activation detectors is by using 3 inch diameter copper samples in a 6” NaI coincidence system. This measurement gave the most accurate results relative to the lead probe and reference samples for all the copper samples tested. Furthermore, we found that the total neutron yield as measured with the 3 inch diameter copper samples in the 6” diameter NaI systems is approximately 89 ± 10 % the total neutron yield as measured using the lead, praseodymium and silver detectors. -
Radioactive Isotopes of Copper 578 (1936)
1110 NATURE jUNE 26, 1937 axis, no water is being frozen or melted with change 10h.l,5.8• Madsen has directed attention to the in temperature. When the curve is vertical with confusion over these periods and gives the half-life respect to the temperature axis, pure water is being period of the copper obtained from zinc as 17h!. frozen or melted. When the curve is inclined to the Leo Szilard, of the Clarendon Laboratory, Oxford, temperature axis, ice is being separated from or informed us that the decay curve of the copper melted into a solution, as in the concentration or bombarded by fast neutrons from radon-beryllium dilution of a sugar solution. This latter effect may contained a period of a few hours which was absent also be brought about by capillary forces or by col when slow neutrons were used, or if the radioactive loidal substances the avidity of which for water copper was obtained from zinc by fast neutron varies with their concentration. bombardment. It was arranged with him that Fig. 3 shows the results obtained upon freezing further investigations and chemical separation should fresh green peas using time as a variable instead of be made in this laboratory. temperature. 19 gm. of green peas at room tempera We irradiated 2 gm. mols of pure cupric oxide with ture were placed in the condenser and immersed in a fast neutrons from a 200 me. radium-beryllium thermostat held at -16° C. At the time of immersion source. After irradiation, we dissolved the oxide, and at intervals thereafter capacitance measurements added 500 mgm. -
Impact-Ionization Mass Spectrometry of Cosmic Dust
IMPACT-IONIZATION MASS SPECTROMETRY OF COSMIC DUST Thesis by Daniel E. Austin Submitted in Partial Fulfillment of the Requirements for the Degree of Doctor of Philosophy California Institute of Technology Pasadena, California 2003 (Defended November 5, 2002) ii © 2003 Daniel E. Austin All Rights Reserved iii Acknowledgements First of all, I thank Dave Dearden, Professor of Chemistry at Brigham Young University, and a former Caltech graduate student, for encouraging me to pursue graduate work at Caltech. The experiences I gained working with him, both as a research assistant and as a teaching assistant, proved invaluable during my stay here. Numerous people at Caltech have contributed in some way to my research efforts. Foremost among these is my advisor, Jack Beauchamp, who successfully balances providing advice and supervision with the hands-off approach that is essential to developing creativity, resourcefulness, and drive in students. I also thank all the other Beauchamp group members who have helped me in so many ways: Dmitri Kossakovski, Sang-Won Lee, Jim Smith, Heather Cox, Ron Grimm, Ryan Julian, and Rob Hodyss. I joined Jack’s group in part because of the very high caliber of students at that time. It seems I am leaving a group equally outstanding. There could be no finer collection of colleagues than this. Minta Akin, an undergraduate Caltech student, has helped tremendously with the ice accelerator, and I thank her for all her work. Mike Roy, Guy Duremberg, and Ray Garcia, the chemistry department machinists, have been both helpful and patient with me as I’ve built numerous instrument parts. -
Copper Isotope Fractionation During Surface Adsorption And
University of Texas at El Paso DigitalCommons@UTEP Open Access Theses & Dissertations 2010-01-01 Copper Isotope Fractionation During Surface Adsorption And Intracellular Incorporation By Bacteria Jesica Urbina Navarrete University of Texas at El Paso, [email protected] Follow this and additional works at: https://digitalcommons.utep.edu/open_etd Part of the Biogeochemistry Commons, Geochemistry Commons, and the Microbiology Commons Recommended Citation Navarrete, Jesica Urbina, "Copper Isotope Fractionation During Surface Adsorption And Intracellular Incorporation By Bacteria" (2010). Open Access Theses & Dissertations. 2553. https://digitalcommons.utep.edu/open_etd/2553 This is brought to you for free and open access by DigitalCommons@UTEP. It has been accepted for inclusion in Open Access Theses & Dissertations by an authorized administrator of DigitalCommons@UTEP. For more information, please contact [email protected]. COPPER ISOTOPE FRACTIONATION DURING SURFACE ADSORPTION AND INTRACELLULAR INCORPORATION BY BACTERIA JESICA URBINA NAVARRETE Environmental Science Program APPROVED: David Borrok, Ph.D., Chair Jasper G. Konter, Ph.D. Joanne T. Ellzey, Ph.D. Patricia D. Witherspoon, Ph.D. Dean of the Graduate School Copyright by Jesica Urbina Navarrete 2010 COPPER ISOTOPE FRACTIONATION DURING SURFACE ADSORPTION AND INTRACELLULAR INCORPORATION BY BACTERIA by JESICA URBINA NAVARRETE, B.S. THESIS Presented to the Faculty of the Graduate School of The University of Texas at El Paso in Partial Fulfillment of the Requirements for the Degree of MASTER OF SCIENCE Department of Environmental Science THE UNIVERSITY OF TEXAS AT EL PASO August 2010 Acknowledgements This publication was made possible through funding by the National Science Foundation (NSF) grant 0745345, the Center for Earth and Environmental Isotope Research (NSF MRI grant 0820986) and by grant number 2G12RR008124-16A1 from the National Center for Research Resources (NCRR), a component of the National Institutes of Health (NIH). -
Natural Variations of Copper and Sulfur Stable Isotopes in Blood of Hepatocellular Carcinoma Patients
Natural variations of copper and sulfur stable isotopes in blood of hepatocellular carcinoma patients Vincent Baltera,1, Andre Nogueira da Costab, Victor Paky Bondanesea, Klervia Jaouenc, Aline Lambouxa, Suleeporn Sangrajrangd, Nicolas Vincenta, François Fourela, Philippe Télouka, Michelle Gigoue, Christophe Lécuyera,f, Petcharin Srivatanakuld, Christian Bréchote,g, Francis Albarèdea, and Pierre Hainauth,i aUMR 5276, Laboratoire de Géologie de Lyon, École Normale Supérieure de Lyon, CNRS, Université de Lyon 1, BP 7000 Lyon, France; bMechanistic Toxicology & Molecular Pathology Department of Non-Clinical Development, UCB BioPharma, SPRL Chemin du Foriest 1, B-1420 Braine L’Alleud, Belgium; cDepartment of Human Evolution, Max Planck Institute for Evolutionary Anthropology, 04103 Leipzig, Germany; dNational Cancer Institute, Bangkok 10400, Thailand; eUnité 785, Pathogénèse et Traitement de l’Hépatite Fulminante et du Cancer du Foie, INSERM, Université Paris-Sud, 94800 Villejuif, France; fInstitut Universitaire de France, 75005 Paris, France; gInstitut Pasteur, 75015 Paris, France; hUnité 823, Ontogenèse et Oncogenèse Moléculaire, Institut Albert Bonniot, INSERM, Université Joseph Fourier, 38706 Grenoble, France; and iStrathclyde Institute of Global Public Health, International Prevention Research Institute, 69006 Lyon, France Edited by Thure E. Cerling, University of Utah, Salt Lake City, UT, and approved December 22, 2014 (received for review August 7, 2014) The widespread hypoxic conditions of the tumor microenviron- and sulfide (5). Regardless of the element, differences in co- ment can impair the metabolism of bioessential elements such as ordination and redox states are generally associated with isotopic copper and sulfur, notably by changing their redox state and, as effects known as isotopic fractionation. In animal models, the a consequence, their ability to bind specific molecules. -
Endf-201 Enof/B-Vi Summary Documentation
BNL-NCS—1754Z DE92 010079 ENDF-201 ENOF/B-VI SUMMARY DOCUMENTATION Compiled and Edited by P.F. Rose Brookhaven National Laboratory October 1991 NATIONAL NUCLEAR DATA CENTER BROOKHAVEN NATIONAL LABORATORY ASSOCIATED UNIVERSITIES, INC. UPTON, LONG ISLAND, NEW YORK 11973 ft UNDER CONTRACT NO. DE-AC02-76CH00016 WITH THE UNITED STATES DEPARTMENT OF ENERGY »'•'—••-.-*>„.,, DISCLAIMER This report was prepared as an account of work sponsored by an agency of the United States Government. Neither the United States Government nor any agency thereof, nor any of their employees, nor any of their contractors, subcontractors, or their employees, makes any warranty, express or implied, or assumes any legal liability or responsibility for the accuracy, completeness, or usefulness of any information, apparatus, product, or process disclosed, or represents that its use would not infringe privately owned rights. Reference herein to any specific commercial product, process, or service by trade name, trademark, manufacturer, or otherwise, does not necessarily constitute or imply its endorsement, recommendation, or favoring by the United States Government or any agency, contractor or subcontractor thereof. The views and opinions of authors expressed herein do not necessarily state or reflect those of the United States Government or any agency, contractor or subcontractor thereof. Printed in the United States of America Available from National Technical Information Service U.S. Department of Commerce 5285 Port Royal Road Springfield, VA 22161 NTIS price codes: -
Isotopic Composition of Some Metals in the Sun
SNSTITUTE OF THEORETICAL ASTROPHYSICS BLINDERN - OSLO REPORT .No. 35 ISOTOPIC COMPOSITION OF SOME METALS IN THE SUN by ØIVIND HAUGE y UNIVERSITETSFORLAqET • OSLO 1972 Universitetsfc lagets trykningssentral, Oslo INSTITUTE OF THEORETICAL ASTROPHYSICS BLINDERN - OSLO REPORT No. 35 ISOTOPIC COMPOSITION OF SOME METALS IN THE SUN by ØIVIND HAUGE UNIVERSITETSFORLAGET • OSLO 1972 Universitetsforlagets tryknlngssentral, Oslo CONTENTS Abstract 1 1. Introduction 2 2. Fine structure in spectral lines from atoms 5 1. Isotope shift 5 2. Hyperfine structure 6 3. Applications to atomic lines in photospheric spectrum .... 8 1. Elements with one odd isotope , 9 2. Elements with two odd isotopes 9 3. Elements with one odd and several even isotopes 11 k. Elements with several odd and even isotopes 11 h. Studies of elements in the Sun with two odd isotopes 1. Isotopes of rubidium 12 A. Observations lk B. Calculations 16 C. The Rb I line at 78OO Å 1. The continuum level 16 2. Line profiles and turbulent velocities 18 3. The asymmetry of the Si I line 19 h. Isotope investigations 21 P. The Rb I line at 79^7 A 28 E. The isotope ratio of rubidium 31 F. The abundance of rubidium 3k 2. Isotopes of antimony 35 A. Spectroscopic data 35 B. The Sb I lines at 3267 and 3722 A 37 3* Isotopes of europium 1*0 A. Observations and methods of analysis ^1 B. Spectroscopic data 1*1 C. Spectral line investigations 1. Investigations of four Eu II lines **3 2. The Eu II lines at Ul29 and U205 k ^6 D. The isotope ratio of europium 50 E. -
ME 120 Lecture Notes: Basic Electricity Fall 2013 This Course Module Introduces the Basic Physical Models by Which We Explain the Flow of Electricity
ME 120 Lecture Notes: Basic Electricity Fall 2013 This course module introduces the basic physical models by which we explain the flow of electricity. The Bohr model of an atom – a nucleus surrounded by shells of electrons traveling in discrete orbits – is presented. Electrical current is described as a flow of electrons. Ohm’s law – the relationship between voltage, current and electrical resistance – is introduced. Learning Objectives Understanding the basic principles of electricity is a foundational skill for all branches of engineering. Understanding electricity is required to use electrical sensors, switches, digital and analog devices, and microcontrollers. In this course, students will use breadboards to assemble electronic components for reading data from sensors and controlling switches, lights, motors and other actuators. The larger purpose is to learn how to incorporate electronic components into solutions to engineering design problems. Successful completion of this module will enable students to 1. Link basic model of an atom to the flow of electricity; 2. Understand the definition of fundamental quantities related to the flow of electricity: coulomb, amp, volt, ohm, joule and watt; 3. Apply Ohm’s Law to single electronic components and to simple circuits. Definition of Electricity and Electrical Charge According to the Concise Oxford English Dictionary, revised 10th edition, the first definition of electricity is a form of energy resulting from the existence of charged particles (such as electrons or protons), either statically as an accumulation of charge or dynamically as a current. Thus, to describe electricity we must introduce the idea of positive and negative electrical charge, which is stored energy associated with particles – electrons and protons. -
Some Basic Concepts
Topics SI units Protons, electrons and neutrons Elements and allotropes States of matter Atomic number 1 Isotopes Relative atomic mass The mole Some basic Gas laws The periodic table concepts Radicals and ions Molecules and compounds Solution concentration Stoichiometry Oxidation and reduction Basic nomenclature 1.1 What is chemistry and why is it important? Matter, be it animal, vegetable or mineral, is composed of chemical elements or combinations thereof. Over a hundred elements are known, although not all are abundant by any means. The vast majority of these elements occur naturally, but some, such as technetium and curium, are artificial. Chemistry is involved with the understanding of the properties of the elements, how they interact with one another, and how the combination of these elements gives compounds that may undergo chemical changes to generate new compounds. Life has evolved systems that depend on carbon as a fundamental element; carbon, hydrogen, nitrogen and oxygen are particularly important in biological systems. A true understanding of biology and molecular biology must be based upon a full knowledge of the structures, properties and reactivities of the molecular components of life. This basic knowledge comes from the study of chemistry. The line between physical and chemical sciences is also a narrow one. Take, for example, the rapidly expanding field of superconducting materials – compounds that possess negligible resistance to the flow of electrons. Typically, this property persists only at very low temperatures but if the super- " conducting materials are to find general application, they must operate at Superconductors: ambient temperatures. Although a physicist may make the conductivity meas- see Box 9.4 urements, it is the preparation and study of the chemical composition of the materials that drive the basic research area. -
Chemistry I Isotope Practice 1. Iron Has Four Naturally Occurring
Chemistry I Isotope Practice GET IN THE HABIT OF SHOWING ALL WORK (I MEAN THAT!) 1. Iron has four naturally occurring isotopes: Fe-54, 53.9396 amu, 5.82% Fe-56, _______ amu, ____% Fe-57, 56.9354 amu, 2.19% Fe-58, 57.9333 amu, 0.33% Determine the mass (rounded to the 0.0001amu) and abundance (rounded to the 0.00%) of Fe-56. 2. Boron has two naturally occurring isotopes: B-10, 10.0129 amu B-11, 11.0093 amu Determine the abundance (rounded to the 0.00%) of each isotope. 3. Silicon has three naturally occurring isotopes: Si-28, _______ amu, ____% Si-29, 28.97649 amu, 4.70% Si-30, 29.97376 amu, 3.09% Determine the mass (rounded to the 0.00001amu) and abundance (rounded to the 0.00%) of Si-28. 4. Antimony has two naturally occurring isotopes: Sb-121, 120.9038 amu Sb-123, 122.9041 amu Determine the abundance (rounded to the 0.00%) of each isotope. 5. Bromine has two naturally occurring isotopes: Br-79, 78.9183 amu, 50.54% Br-81, _______ amu, ____% Determine the mass (rounded to the 0.0001amu) and abundance (rounded to the 0.00%) of Br-81. 6. Silver has two naturally occurring isotopes: Ag-107, 106.90509 amu Ag-109, 108.90470 amu Determine the abundance (rounded to the 0.00%) of each isotope. Chemistry I Isotopes, Moles, Representative Particles, and Mass Practice GET IN THE HABIT OF SHOWING ALL WORK (I MEAN THAT!) 1. Neon has three naturally occurring isotopes: Ne-20, 19.99244amu, 90.92% Ne-21, 20.99395amu, 0.26% Ne-22, _______amu, ____% Determine the mass (rounded to the 0.00001amu) and abundance (rounded to the 0.00%) of Ne-22. -
Copper Isotope Constraints on the Genesis of the Keweenaw Peninsula Native Copper District, Michigan, USA
minerals Article Copper Isotope Constraints on the Genesis of the Keweenaw Peninsula Native Copper District, Michigan, USA Theodore J. Bornhorst 1,* ID and Ryan Mathur 2 1 A. E. Seaman Mineral Museum and Department of Geological and Mining Engineering and Sciences, Michigan Technological University, Houghton, MI 49931, USA 2 Department of Geology, Juniata College, Huntingdon, PA 16652, USA; [email protected] * Correspondence: [email protected]; Tel.: +1-906-487-2721 Received: 22 August 2017; Accepted: 20 September 2017; Published: 30 September 2017 Abstract: The Keweenaw Peninsula native copper district of Michigan, USA is the largest concentration of native copper in the world. The copper isotopic composition of native copper was measured from stratabound and vein deposits, hosted by multiple rift-filling basalt-dominated stratigraphic horizons over 110 km of strike length. The δ65Cu of the native copper has an overall mean of +0.28‰ and a range of −0.32‰ to +0.80‰ (excluding one anomalous value). The data appear to be normally distributed and unimodal with no substantial differences between the native copper isotopic composition from the wide spread of deposits studied here. This suggests a common regional and relatively uniform process of derivation and precipitation of the copper in these deposits. Several published studies indicate that the ore-forming hydrothermal fluids carried copper as Cu1+, which is reduced to Cu0 during the precipitation of native copper. The δ65Cu of copper in the ore-forming fluids is thereby constrained to +0.80‰ or higher in order to yield the measured native copper values by reductive precipitation. The currently accepted hypothesis for the genesis of native copper relies on the leaching of copper from the rift-filling basalt-dominated stratigraphic section at a depth below the deposits during burial metamorphism.