Synthesis of Analogues of Dicoumarol and Their Measurement As Inhibitors of NQO1
Total Page:16
File Type:pdf, Size:1020Kb
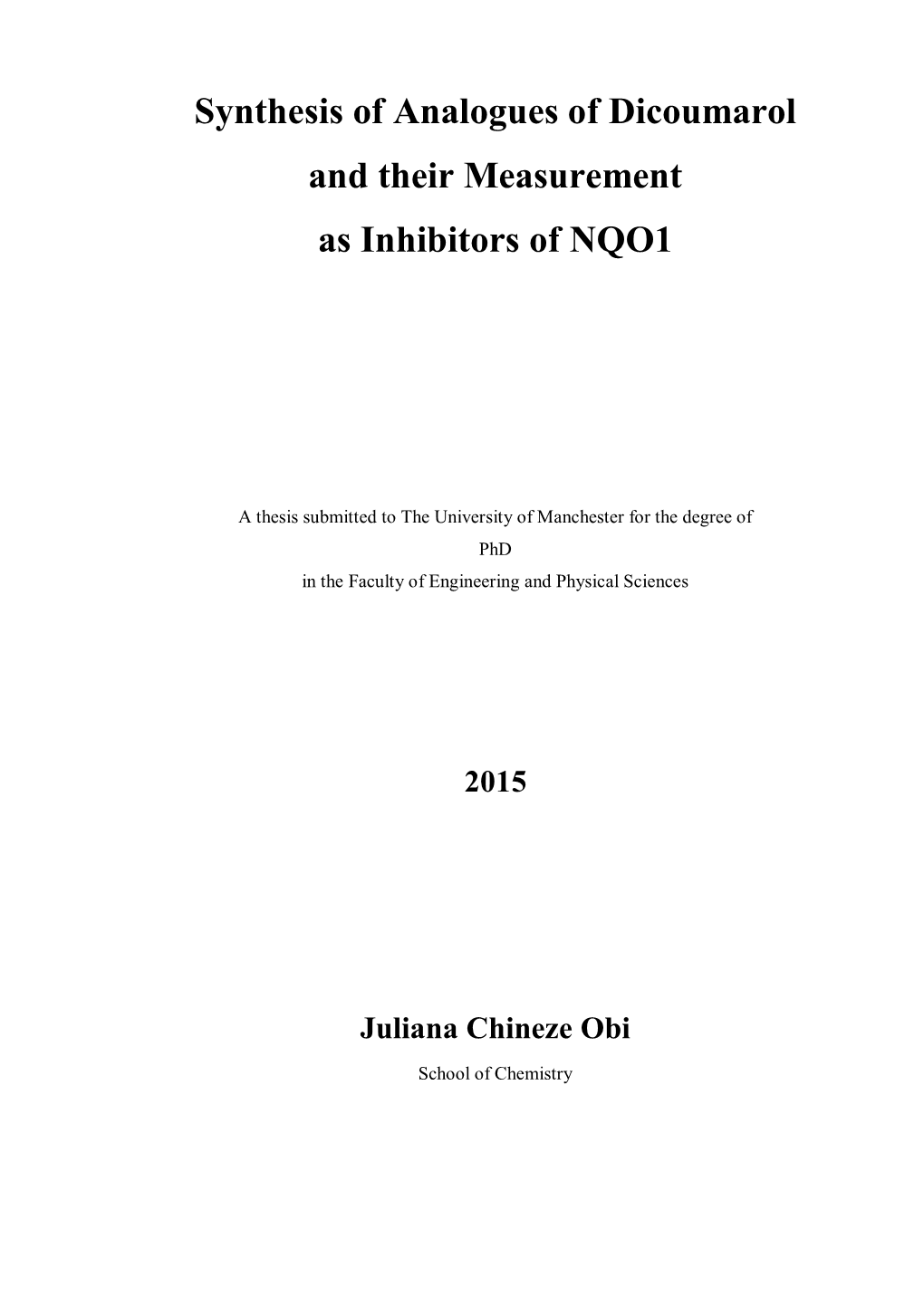
Load more
Recommended publications
-
Occurrence, Elimination, and Risk of Anticoagulant Rodenticides in Wastewater and Sludge
Occurrence, elimination, and risk of anticoagulant rodenticides in wastewater and sludge Silvia Lacorte, Cristian Gómez- Canela Department of Environmental Chemistry, IDAEA-CSIC, Jordi Girona 18-26, 08034 Barcelona Rats and super-rats Neverending story 1967 Coumachlor 1 tn rodenticides /city per campaign “It will be the LAST ONE” Rodenticides Biocides: use regulated according to EU. Used mainly as bait formulations. First generation: multiple feedings, less persistent in tissues, commensal and outdoor use. Second generation: single feeding (more toxic), more persistent in tissue, commensal use only. Toxic: vitamin K antagonists that cause mortality by blocking an animal’s ability to produce several key blood clotting factors. High oral, dermal and inhalation toxicity. Origin and fate of rodenticides Study site: Catalonia (7.5 M inhabitants) 1693 km of sewage corridor 13 fluvial tanks (70.000 m3) 130,000,000 € / 8 YEARS 32,000 km2 378,742 kg/y AI 2,077,000 € Objectives 1. To develop an analytical method to determine most widely used rodenticides in wastewater and sludge. 2. To monitor the presence of rodenticides within 9 WWTP receiving urban and agricultural waters. 3. To evaluate the risk of rodenticides using Daphnia magna as aquatic toxicological model. 4. To study the accumulation of rodenticides in sludge. Compounds studied Coumachlor* Pindone C19H15ClO4 C14H14O3 Dicoumarol Warfarin C19H12O6 C19H16O4 Coumatetralyl Ferulenol FGARs C19H16O3 C24H30O3 Acenocoumarol Chlorophacinone • Solubility C19H15NO6 C23H15ClO3 0.001-128 mg/L • pKa 3.4-6.6 Flocoumafen Bromadiolone C H F O C H BrO 33 25 3 40 30 23 4 • Log P 1.92-8.5 Brodifacoum Fluindione C H BrO 31 23 3 C15H9FO2 SGARs Difenacoum Fenindione C31H24O3 C15H10O2 1. -
New Zealand Data Sheet 1
New Zealand Data Sheet 1. PRODUCT NAME NAXEN® 250 mg tablets 2. QUALITATIVE AND QUANTITATIVE COMPOSITION Each NAXEN 250 mg tablet contains 250 mg of Naproxen For the full list of excipients, see section 6.1. 3. PHARMACEUTICAL FORM NAXEN 250 mg tablets are yellow, biconvex, round tablet of 11 mm diameter with one face engraved NX250 and having a bisecting score. The score line is only to facilitate breaking for ease of swallowing and not to divide into equal doses. 4. CLINICAL PARTICULARS 4.1. Therapeutic indications NAXEN is indicated in adults for the relief of symptoms associated with rheumatoid arthritis, osteoarthritis, ankylosing spondylitis, tendonitis and bursitis, acute gout and primary dysmenorrhoea. NAXEN is indicated in children for juvenile arthritis. 4.2. Dose and method of administration After assessing the risk/benefit ratio in each individual patient, the lowest effective dose for the shortest possible duration should be used (see Section 4.4). During long-term administration the dose of naproxen may be adjusted up or down depending on the clinical response of the patient. A lower daily dose may suffice for long-term administration. In patients who tolerate lower doses well, the dose may be increased to 1000 mg per day when a higher level of anti-inflammatory/analgesic 1 | P a g e activity is required. When treating patients with naproxen 1000 mg/day, the physician should observe sufficient increased clinical benefit to offset the potential increased risk. Dose Adults For rheumatoid arthritis, osteoarthritis and ankylosing spondylitis Initial therapy: The usual dose is 500-1000 mg per day taken in two doses at 12 hour intervals. -
Perioperative Management of Patients Treated with Antithrombotics in Oral Surgery
SFCO/Perioperative management of patients treated with antithrombotic agents in oral surgery/Rationale/July 2015 SOCIÉTÉ FRANÇAISE DE CHIRURGIE ORALE [FRENCH SOCIETY OF ORAL SURGERY] IN COLLABORATION WITH THE SOCIÉTÉ FRANÇAISE DE CARDIOLOGIE [FRENCH SOCIETY OF CARDIOLOGY] AND THE PERIOPERATIVE HEMOSTASIS INTEREST GROUP Space Perioperative management of patients treated with antithrombotics in oral surgery. RATIONALE July 2015 P a g e 1 | 107 SFCO/Perioperative management of patients treated with antithrombotic agents in oral surgery/Rationale/July 2015 Abbreviations ACS Acute coronary syndrome(s) ADP Adenosine diphosphate Afib Atrial Fibrillation AHT Arterial hypertension Anaes Agence nationale d’accréditation et d’évaluation en santé [National Agency for Accreditation and Health Care Evaluation] APA Antiplatelet agent(s) aPTT Activated partial thromboplastin time ASA Aspirin BDMP Blood derived medicinal products BMI Body mass index BT Bleeding Time cAMP Cyclic adenosine monophosphate COX-1 Cyclooxygenase 1 CVA Cerebral vascular accident DIC Disseminated intravascular coagulation DOA Direct oral anticoagulant(s) DVT Deep vein thrombosis GEHT Study Group on Hemostasis and Thrombosis (groupe d’étude sur l’hémostase et la thrombose) GIHP Hemostasis and Thrombosis Interest Group (groupe d’intérêt sur l’hémostase et la thrombose) HAS Haute autorité de santé [French Authority for Health] HIT Heparin-induced thrombocytopenia IANB Inferior alveolar nerve block INR International normalized ratio IV Intravenous LMWH Low-molecular-weight heparin(s) -
Drug Interactions and Lethal Drug Combinations
J Clin Pathol: first published as 10.1136/jcp.28.Suppl_9.94 on 1 January 1975. Downloaded from J. clin. Path., 28, Suppl. (Roy. Coll. Path.), 9, 94-98 The drug dilemma-benefits and hazards Drug interactions and lethal drug combinations ALAN RICHENS From the Department of Clinical Pharmacology, St Bartholomew's Hospital, London Although the development of drugs of greater example, aspirin can displace oral anticoagulants potency and efficacy confers on the physician from their plasma-protein-binding sites, and in- increasing power to treat serious diseases, it also directly acting sympathomimetics contained in cough increases the number and seriousness of potential mixtures can cause a hypertensive crisis in patients adverse effects and drug interactions which can on monoamine oxidase inhibitors. Self-medication is occur. Most hospital patients receive more than one common, and often involves drugs obtained on drug at a time, the average number often being prescription for a previous illness. greater than five (Smith, Seidl, and Cluff, 1966). The 4 When several clinics or doctors are involved in incidence of drug reactions rises with the number of the care of a patient, one doctor may not be aware of drugs prescribed simultaneously. In patients pre- what another has prescribed. scribed one to five drugs the incidence of reactions is 5 When preparations which contain more than 18-6%, while in patients prescribed six or more one ingredient are prescribed by their trade names. it rises to 814 % (Hurwitz and Wade, 1969). There are a number of ways in which drugs may The Boston Collaborative Drug Surveillance interact. -
Dicoumarol: a Unique Microtubule Stabilizing Natural Product That Is Synergistic with Taxol1
[CANCER RESEARCH 63, 1214–1220, March 15, 2003] Dicoumarol: A Unique Microtubule Stabilizing Natural Product that Is Synergistic with Taxol1 Hamta Madari, Dulal Panda, Leslie Wilson, and Robert S. Jacobs2 Departments of Ecology, Evolution, and Marine Biology [H. M., R. S. J.] and Molecular, Cellular, and Developmental Biology [L. W.], University of California, Santa Barbara, California 93106, and Bhupat and Jyoti Mehta School of Biosciences and Bioengineering, Indian Institute of Technology, Bombay, Mumbai, India 400076 [D. P.] ABSTRACT prostate cancer, malignant melanoma, and metastatic renal cell carci- noma (12–14). In studies on the antiproliferative actions of coumarin compounds, we In addition, the coumarin anticoagulants, dicoumarol (Dicumarol) -discovered that dicoumarol (a coumarin anticoagulant; 3,3-methyl and its synthetic derivative warfarin sodium (Coumadin), have been enebis[4-hydroxycoumarin]) inhibits the first cleavage of Strongylocentro- tus purpuratus (sea urchin) embryos in a concentration-dependent manner shown to decrease metastases in experimental animals (15). Warfarin with 50% inhibition occurring at a concentration of 10 M. Because first sodium, largely replacing dicoumarol therapeutically as an anticoag- cleavage in sea urchin embryos is highly selective for microtubule- ulant, has been used for the treatment of a variety of cancers and targeted agents, we thought that the active compounds might inhibit cell shown to improve tumor response rates and survival in patients with division by interacting with tubulin or microtubules. We found that several types of cancer (16–22). However, despite numerous studies, dicoumarol binds to bovine brain tubulin with a Kd of 22 M and that 0.1 little information has been acquired on the cellular mechanism of M dicoumarol strongly stabilizes the growing and shortening dynamics action of coumarin compounds in the treatment of malignancies. -
Some Aspects of the Pharmacology of Oral Anticoagulants
Some aspects of the pharmacology of oral anticoagulants The pharmacology of oral anticoagulants ls discussed with particular rejerence to data of value in the management of therapy. The importance of individual variability in response and drug interaction is stressed. Other effects of these agents which may have clinical utility are noted. William W. Coon, M.D., and Park W. Willis 111, M.D., Ann Arbor, Mich. The Departments of Surgery (Section of General Surgery) and Medicine, University of Michigan Medical School In the twenty-five years sinee the isola dividual struetural features but by a com tion of the hemorrhagie faetor in spoiled bination of several: molecular shape, in sweet clever," the gradually inereasing creased aetivity with 6 membered hetero utilization of oral antieoagulants for the eyclic rings with a substituent in position prevention and therapy of thromboembolie 8 and with a methoxyl rather than a free disease has made them one of the most hydroxl group. Also important is the dernon widely used groups of pharmacologic stration that levorotatory warfarin is seven agents. This review is restrieted to as times more aetive than its enantiomer.F" peets of the pharmaeology of these agents As Hunter and Shepherd'" have pointed whieh may be important to their proper out, the failure to obtain a precise cor clinieal utilization. relation between strueture and antieoagu lant aetivity is "not surprising in view of Relation of structure to function the influence of small struetural changes The oral antieoagulants have been di on sueh variables as solubility, rate of ab vided into four main groups on the basis sorption, ease of distribution, degree of of ehemieal strueture (Fig. -
Perioperative Management of Patients Receiving OAC Or Anti-Platelet
Perioperative Management of Patients Receiving Oral Anticoagulants or Antiplatelet Agents Brett C Stoll, MD, FACC February 28, 2015 Disclosures • None related to this presentation Case #1 • 78 yo man with proximal LAD stent (DES) 2 weeks ago, presents with displaced hip fracture. Peri-procedural management? – Hold both aspirin and Plavix for 7-10 days, resume post- procedure – Hold Plavix only for 7-10 days, then resume post- procedure – Inpatient Integrilin bridge Case #2 • 53yo man with history of an MI and a stent 2 years ago is scheduled for routine screening colonoscopy. Peri-procedural management? – Hold both aspirin and Plavix for 7-10 days, resume post- procedure – Hold Plavix only for 7-10 days, then resume post- procedure – Inpatient Integrilin bridge Case #3 • 70 yo man with chronic a-fib and admitted for colonoscopy. Coumadin was held prior to the procedure. Post-procedure management? – IV UFH until INR > 2.0 – Outpatient LMWH bridge – Resume Coumadin without a bridge Case #4 • 48 yo woman with bi-leaflet aortic valve is to undergo elective inguinal hernia repair. Post- op management? – IV UFH until INR > 2.0 – Outpatient LMWH bridge – Resume Coumadin without a bridge Case #5 • 70 yo man with a mechanical mitral valve (ball in cage) with a-fib, prior CVA, and undergoes colectomy. Post-op management? – IV UFH until INR > 2.0 – Outpatient LMWH bridge – Resume Coumadin without a bridge Anti-platelet therapy • Anti-platelet agents – Cyclo-oxygenase inhibitor • Aspirin – Adenosine diphosphate receptor (ADP) inhibitors • Clopidogrel (Plavix) • Prasugrel (Effient) • Ticagrelor (Brilinta) Anti-platelet therapy • Anti-platelet agents – Other agents • Phosphodiesterase inhibitors (Cilostazol (Pletal)) • Adenosine reuptake inhibitors (Dipyridamole (Persantine)) • Protease-activated receptor-1 (PAR-1) antagonists (Vorapaxar (Zontivity)) • Glycoprotein IIB/IIIA inhibitors – Abciximab (Reopro) – Eptifibatide (Integrilin) – Tirofiban (Aggrastat) Aspirin • Aspirin is the most widely used drug in medicine. -
Comparing Antithrombotic Strategies After Bioprosthetic
Antithrombotic Strategies after bAVR Evidence-based Synthesis Program APPENDIX A. SEARCH STRATEGIES DATABASES/WEBSITES: Ovid Medline 1946 to June 19, 2017 PubMed (non-Medline materials) January 9, 2017 Elsevier EMBASE February 1, 2017 EBM Reviews (CDSR, DARE, HTA, Cochrane CENTRAL, etc.) January 24, 2017 Clinicaltrials.gov January 24, 2017 RoPR (Registry of Patient Registries January 24, 2017 SEARCH STRATEGIES Updated search strategy – 9Jan2017, after adding “placement” based on Stevenson editorial: Epub Ahead of Print, In-Process & Other Non-Indexed Citations, Ovid MEDLINE(R) Daily and Ovid MEDLINE(R) 1946 to Present Date Searched: January 9, 2017 Searched by: Robin Paynter, MLIS 1 Heart Valve Prosthesis/ or Heart Valve Prosthesis Implantation/ or Transcatheter Aortic Valve Replacement/ or 80730 (((aort* or valve*) adj3 (implant* or replac* or graft*)) or AVR or AVRs or mini-AVR* or "surgical AVR*" or SAVR or SAVRs or "bioprosthe* AVR*" or "bio-prosthe* AVR*" or "biologic* AVR*" or bAVR* or TAVI* or TAVR* or PAVR* or ((transcatheter* or trans-catheter* or transfemoral* or trans-femoral* or transapical* or trans-apical* or transaxillar* or trans-axillar* or transvascular* or trans-vascular* or percutaneous* or bioprosthet* or bio-prosthet* or biologic*) adj3 (implant* or placement* or replac* or graft*))).tw,kf. 2 aortic valve/ or (aort* or answer or "Anticoagulation Treatment Influence on Postoperative Patients" or 998641 action).tw,kf. 3 bioprosthesis/ or (bioprosthe* or bio-prosthe* or ((biologic* or tissue* or prosthe*) adj3 (aort* or valv* or graft*)) or 512785 bovine* or porcine* or equine* or xenograft* or xenogen* or heterograft* or xenobioprosthe* or 3F* or ACURATE- TA* or Biocor* or Carpentier-Edwards* or COLIBRI* or CoreValve* or Crown PRT* or DOKIMOS* or Engager* or EPIC* or Freestyle* or FS or HANCOCK* or INSPIRIS* or J-Valve* or JENAVALVE* or MITROFLOW* or MOSAIC* or MYVAL* or Perceval* or Perimount* or Sapien* or SOLO or TLPB* or TRIFECTA*).tw,kf. -
Summary of Product Characteristics
SUMMARY OF PRODUCT CHARACTERISTICS 4 1. NAME OF THE MEDICINAL PRODUCT Revasc 2. QUALITATIVE AND QUANTITATIVE COMPOSITION One vial of Revasc contains 15 mg desirudin (INN) corresponding to approximately 270 000 antithrombin units (ATU) or 18 000 ATU per mg of desirudin with reference to the WHO Second International Standard for α-thrombin. Desirudin is a recombinant DNA product derived from yeast cells. Desirudin is a single chain polypeptide consisting of 65 amino acid residues and 3 disulphide bridges. Vials of desirudin are supplied with solvent ampoules containing pyrogen-free Mannitol Ph. Eur. in Water for injections Ph. Eur. 3. PHARMACEUTICAL FORM Powder for injection to be reconstituted prior to subcutaneous injection with 0.5 ml mannitol solvent for solution (3%) which is supplied with the product. 4. CLINICAL PARTICULARS 4.1 THERAPEUTIC INDICATIONS Prevention of deep venous thrombosis in patients undergoing elective hip and knee replacement surgery. 4.2 POSOLOGY AND METHOD OF ADMINISTRATION Adult and elderly patients The recommended dose is 15 mg twice daily. The first injection should be initiated 5 to 15 minutes before surgery but after induction of regional block anaesthesia, if used. Treatment with desirudin is then continued twice daily post-operatively for 9 to a maximum of 12 days or until the patient is fully ambulant, whichever occurs first. Currently, there is no clinical experience to support the use of desirudin beyond 12 days. Administration is by subcutaneous injection, preferably at an abdominal site. Injections should be rotated between at least four different sites. Children There is no clinical experience with desirudin in children. -
Evidence-Based Practice Center Systematic Review Protocol
Evidence-based Practice Center Systematic Review Protocol Project Title: Comparative Effectiveness of Pharmacologic and Mechanical Prophylaxis of Venous Thromboembolism Among Special Populations Background Prevalence of Venous Thromboembolism Pulmonary embolism (PE) resulting from deep vein thrombosis (DVT), collectively known as venous thromboembolism (VTE), affects an estimated 900,000 Americans each year and results in significant morbidity and mortality.1,2 The average annual incidence of DVT in the United States ranges from 48 to 122 per 100,000.1,2 With the ageing U.S. population, the number of cases of VTE is likely to rise. Adverse Consequences of Venous Thromboembolism There are significant adverse consequences of DVT and PE.1 Two-thirds of all VTE cases are nonfatal and result in hundreds of thousands of hospitalizations, whereas approximately one-third of these cases are fatal and result in an estimated 300,000 deaths each year.1,2 The cost of hospitalization for another medical condition has been shown to increase with the diagnosis of DVT (approximately $10,000) or PE ($20,000).3 Thus VTE is an important patient safety issue that results in significant morbidity, mortality, and health care cost.4 Accordingly, the comparative effectiveness and safety of interventions for the prevention and treatment of VTE are among the national priorities for comparative effectiveness research.5 Pharmacologic Agents and Medical Devices Used for Thromboprophylaxis There are a number of antithrombotic drugs and antithrombotic mechanical devices that are approved by the U.S. Food and Drug Administration (FDA) for various indications. A small proportion of these are approved for primary prophylaxis of VTE, but others may be considered or used off label for this purpose (Table 1). -
Warfarin: from Rat Poison to Clinical
MILESTONES Alumni Research Foundation (WARF), who were awarded the patent for dicoumarol in 1941. In 1945, Link considered using a coumarin derivative as a rodenticide. Dicoumarol acted too slowly to be a practical poison. Link and colleagues worked through a list of 150 varia- imageBROKER / Alamy Stock Photo Stock Alamy / imageBROKER tions of coumarin, and number 42 was found to be particularly potent. The compound was named ‘warfarin’ after the funding agency, and was successfully marketed in 1948 as a rodenticide. In 1951, a US Army inductee attempted suicide with multiple doses of warfarin in rodenticide, but fully recovered after being treated with vitamin K in hospital. Studies then began on the use of warfarin as a therapeutic anticoagulant. Clinical anticoagulants were available at this time, but heparin required parenteral MILESTONE 2 administration, and dicoumarol had a long lag period before onset of a therapeutic effect. The main Warfarin: from rat poison advantages of warfarin were high oral bioavailability and high water solubility; it was more potent than to clinical use dicoumarol, but its effect could still be reversed by vitamin K. Therefore, The anticoagulant drug warfarin fresh blood into the bleeding animal. warfarin transitioned into clinical is widely used to prevent and treat Roderick referred to the acquired use under the trade name Coumadin, deep-vein thrombosis and pul- coagulation disorder as a ‘plasma Warfarin and was approved for use in humans monary embolism, and to prevent prothrombin defect’. transitioned in 1954. stroke in patients who have atrial Despite recommendations not to into clinical An early recipient of warfarin was fibrillation, valvular heart disease, or feed mouldy hay to their cattle, many US president Dwight D. -
The 50-Year Quest to Replace Warfarin Jeffrey Weitz
The 50-year quest to replace warfarin Jeffrey Weitz Anticoagulants are used for the prevention and treatment of venous and arterial to check that the appropriate level of anticoagulation is reached and maintained in thrombosis, the leading cause of morbidity and mortality in the Western world. patients receiving warfarin. Consequently, warfarin is underused, and the level of Warfarin — the prototype oral anticoagulant — is a vitamin K antagonist that has been anticoagulation is often suboptimal even when it is administered. These drawbacks in clinical use since the 1950s. Although they are effective, vitamin K antagonists have highlight the need for new oral anticoagulants. The first drug to be approved — in 2010 DRUG several drawbacks, the most notable of which is the propensity to cause bleeding. — as an alternative to warfarin was dabigatran, a direct thrombin inhibitor. Clinical Other limitations include a slow onset of action, interactions with multiple other drugs studies of the direct factor Xa inhibitors rivaroxaban and apixaban have been DISCOVERY and interpatient variability in drug response. As a result, regular monitoring is required completed and could form the basis for regulatory approval as alternatives to warfarin. Comparative pharmacology of new oral anticoagulants compared to warfarin Venous and arterial thrombosis 'PFQVJGNKWO 8GUUGNYCNN Thrombotic disorders The coagulation cascade Warfarin Dabigatran Rivaroxaban Apixaban Edoxaban Arterial thrombosis. The primary trigger of Prodrug No Yes No No No (KDTKP Pulmonary This is classically divided into three pathways. The extrinsic pathway (also known as the Bioavailability Over 90% 6% 80% 60% 50% this is rupture of an atherosclerotic plaque, embolism ▶ tissue factor pathway) initiates coagulation.