Neuronal Synchronization and Thalamocortical Rhythms During
Total Page:16
File Type:pdf, Size:1020Kb
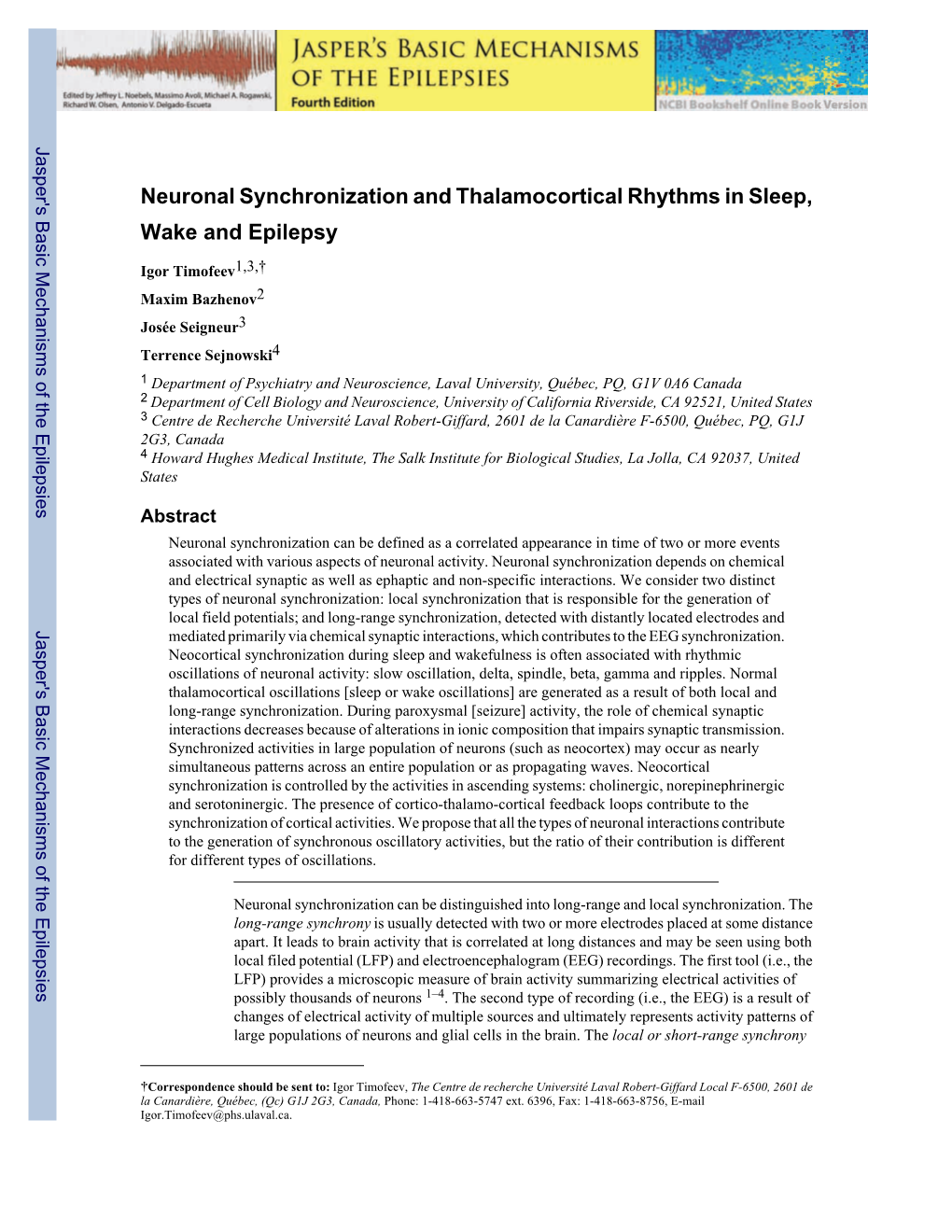
Load more
Recommended publications
-
Learning Alters Theta-Nested Gamma Oscillations in Inferotemporal Cortex
Learning alters theta-nested gamma oscillations in inferotemporal cortex Keith M Kendrick1, Yang Zhan1, Hanno Fischer1 Ali U Nicol1 Xuejuan Zhang 2 & Jianfeng Feng3 1Cognitive and Behavioural Neuroscience, The Babraham Institute, Cambridge, CB22 3AT, UK 2Mathematics Department, Zhejiang Normal University, Jinhua 321004, Zhejian Province, PR China 3Department of Computer Science, Warwick University, Coventry CV4 7AL UK and Centre for Computational Systems Biology, Fudan University, Shanghai, PR China. Corresponding authors: [email protected] [email protected] 1 How coupled brain rhythms influence cortical information processing to support learning is unresolved. Local field potential and neuronal activity recordings from 64- electrode arrays in sheep inferotemporal cortex showed that visual discrimination learning increased the amplitude of theta oscillations during stimulus presentation. Coupling between theta and gamma oscillations, the theta/gamma ratio and the regularity of theta phase were also increased, but not neuronal firing rates. A neural network model with fast and slow inhibitory interneurons was developed which generated theta nested gamma. By increasing N-methyl-D-aspartate receptor sensitivity similar learning-evoked changes could be produced. The model revealed that altered theta nested gamma could potentiate downstream neuron responses by temporal desynchronization of excitatory neuron output independent of changes in overall firing frequency. This learning-associated desynchronization was also exhibited by inferotemporal cortex neurons. Changes in theta nested gamma may therefore facilitate learning-associated potentiation by temporal modulation of neuronal firing. The functions of both low and high frequency oscillations in the brain have been the subject of considerable speculation1. Low frequency theta oscillations (4-8Hz) have been observed to increase in terms of power and phase-locked discharge of single neurons in a visual memory task2. -
Measuring Sleep Quality from EEG with Machine Learning Approaches
Measuring Sleep Quality from EEG with Machine Learning Approaches Li-Li Wang, Wei-Long Zheng, Hai-Wei Ma, and Bao-Liang Lu∗ Center for Brain-like Computing and Machine Intelligence Department of Computer Science and Engineering Key Laboratory of Shanghai Education Commission for Intelligent Interaction and Cognitive Engineering Brain Science and Technology Research Center Shanghai Jiao Tong University, Shanghai, 200240, China Abstract—This study aims at measuring last-night sleep quality Index [2], Epworth Sleep Scale [3], and Sleep Diaries [4]. from electroencephalography (EEG). We design a sleep ex- However, we have no way of knowing whether the respondents periment to collect waking EEG signals from eight subjects are honest, conscientious, responsible for self assessment and under three different sleep conditions: 8 hours sleep, 6 hours sleep, and 4 hours sleep. We utilize three machine learning related records and in many cases they may provide false approaches, k-Nearest Neighbor (kNN), support vector machine information or fill in the questionnaire not seriously. Secondly, (SVM), and discriminative graph regularized extreme learning the subjective evaluation method is more troublesome, time- machine (GELM), to classify extracted EEG features of power consuming and laborious, which requires participants to se- spectral density (PSD). The accuracies of these three classi- riously think about, a detailed answer, record relevant infor- fiers without feature selection are 36.68%, 48.28%, 62.16%, respectively. By using minimal-redundancy-maximal-relevance mation in time, in the meantime, experiment personnel also (MRMR) algorithm and the brain topography, the classification need a manual investigation and analysis of the information accuracy of GELM with 9 features is improved largely and in order to give a sleep quality evaluation and judgment of increased to 83.57% in average. -
Impact Factor: 3.958/ICV: 4.10 ISSN: 0976-7908 306 BRAINWAVES AS
Impact factor: 3.958/ICV: 4.10 ISSN: 0976-7908 306 Pharma Science Monitor 8(2), Apr-Jun 2017 PHARMA SCIENCE MONITOR AN INTERNATIONAL JOURNAL OF PHARMACEUTICAL SCIENCES Journal home page: http://www.pharmasm.com BRAINWAVES AS NEUROPHYSIOLOGICAL OUTCOMES IN NEUROSEDATION Nikhil C. Shah1, Srabona Lahiri1 and Dhrubo Jyoti Sen2* 1Bethany Mission School, Near Ashirwad Flat, Behind Hingraj Society, Mehsana-384002, Gujarat, India 2Shri Sarvajanik Pharmacy College, Gujarat Technological University, Arvind Baug, Mehsana-384001, Gujarat, India ABSTRACT Neural oscillation is rhythmic or repetitive neural activity in the central nervous system. Neural tissue can generate oscillatory activity in many ways, driven either by mechanisms within individual neurons or by interactions between neurons. In individual neurons, oscillations can appear either as oscillations in membrane potential or as rhythmic patterns of action potentials, which then produce oscillatory activation of post-synaptic neurons. At the level of neural ensembles, synchronized activity of large numbers of neurons can give rise to macroscopic oscillations, which can be observed in an electroencephalogram. Oscillatory activity in groups of neurons generally arises from feedback connections between the neurons that result in the synchronization of their firing patterns. The interaction between neurons can give rise to oscillations at a different frequency than the firing frequency of individual neurons. A well- known example of macroscopic neural oscillations is alpha activity. Neural oscillations were observed by researchers as early as 1924 (by Hans Berger). More than 50 years later, intrinsic oscillatory behavior was encountered in vertebrate neurons, but its functional role is still not fully understood. The possible roles of neural oscillations include feature binding, information transfer mechanisms and the generation of rhythmic motor output. -
Nutritional and Herbal Therapies for Children and Adolescents
Nutritional and Herbal Therapies for Children and Adolescents A Handbook for Mental Health Clinicians Nutritional and Herbal Therapies for Children and Adolescents A Handbook for Mental Health Clinicians George M. Kapalka Associate Professor, Monmouth University West Long Branch, NJ and Director, Center for Behavior Modifi cation Brick, NJ AMSTERDAM • BOSTON • HEIDELBERG • LONDON NEW YORK • OXFORD • PARIS • SAN DIEGO SAN FRANCISCO • SINGAPORE • SYDNEY • TOKYO Academic Press is an imprint of Elsevier Academic Press is an imprint of Elsevier 32 Jamestown Road, London NW1 7BY, UK 30 Corporate Drive, Suite 400, Burlington, MA 01803, USA 525 B Street, Suite 1900, San Diego, CA 92101-4495, USA Copyright © 2010 Elsevier Inc. All rights reserved No part of this publication may be reproduced, stored in a retrieval system or transmitted in any form or by any means electronic, mechanical, photocopying, recording or otherwise without the prior written permission of the publisher. Permissions may be sought directly from Elsevier’s Science & Technology Rights Department in Oxford, UK: phone (44) (0) 1865 843830; fax (44) (0) 1865 853333; email: [email protected]. Alternatively, visit the Science and Technology Books website at www.elsevierdirect.com/rights for further information Notice No responsibility is assumed by the publisher for any injury and/or damage to persons or property as a matter of products liability, negligence or otherwise, or from any use or operation of any methods, products, instructions or ideas contained in the material -
Machine Learning Techniques for Detection of Nocturnal Epileptic
Università degli Studi di Cagliari PHD DEGREE Industrial Engineering Cycle XXX Machine Learning Techniques for Detection of Nocturnal Epileptic Seizures from Electroencephalographic Signals Scientific Disciplinary Sector ING-IND/31 PhD Student: Barbara Pisano Coordinator of the PhD Programme Prof. Francesco Aymerich Supervisor Prof. Alessandra Fanni Final exam. Academic Year 2016 – 2017 Thesis defence: March 2018 Session Università degli Studi di Cagliari PHD DEGREE Industrial Engineering Cycle XXX Machine Learning Techniques for Detection of Nocturnal Epileptic Seizures from Electroencephalographic Signals Scientific Disciplinary Sector ING-IND/31 PhD Student: Barbara Pisano Coordinator of the PhD Programme Prof. Francesco Aymerich Supervisor Prof. Alessandra Fanni Final exam. Academic Year 2016 – 2017 Thesis defence: March 2018 Session Barbara Pisano gratefully acknowledges Sardinia Regional Government for the financial support of her PhD scholarship (P.O.R. Sardegna F.S.E. Operational Programme of the Autonomous Region of Sardinia, European Social Fund 2007-2013 - Axis IV Human Resources, Objective l.3, Line of Activity l.3.1.)”. Questa Tesi può essere utilizzata, nei limiti stabiliti dalla normativa vigente sul Diritto d’Autore (Legge 22 aprile 1941 n. 633 e succ. modificazioni e articoli da 2575a 2583 del Codice civile) ed esclusivamente per scopi didattici e di ricerca; è vietato qualsiasi utilizzo per fini commerciali. In ogni caso tutti gli utilizzi devono riportare la corretta citazione delle fonti. La traduzione, l'adattamento totale e parziale, sono riservati per tutti i Paesi. I documenti depositati sono sottoposti alla legislazione italiana in vigore nel rispetto del Diritto di Autore, da qualunque luogo essi siano fruiti. III ACKNOWLEDGEMENTS A few words to thank all the people who made this work possible. -
Thalamocortical Oscillations - Scholarpedia Page 1 of 14
Thalamocortical oscillations - Scholarpedia Page 1 of 14 Thalamocortical oscillations Maxim Bazhenov, The Salk Institute, San Diego, California Igor Timofeev, Laval University, Quebec, Canada Oscillatory activity is an emerging property of the thalamocortical system. The various oscillatory rhythms generated in the thalamocortical system are mediated by two types of mechanisms: intrinsic mechanisms, which depend on the interplay between specific intrinsic currents. extrinsic or network mechanisms, which require the interaction of excitatory and inhibitory neurons within a population. Intrinsic and network mechanisms can work alone (e.g., thalamic delta oscillations depend on the intrinsic properties of thalamic relay cells, cortical slow oscillation depends on network properties) or in combination (e.g., spindles depend on the interaction between thalamic relay and reticular neurons as well as on their intrinsic properties). The patterns and the dominant frequencies of thalamocortical oscillations depend on the functional state of the brain. Oscillations Normal thalamocortical oscillatory activities include infra-slow : 0.02-0.1 Hz, slow : 0.1-15 Hz (present mainly during slow-wave sleep or anesthesia), which are further divided on slow oscillation (0.2-1 Hz), delta (1-4 Hz), spindle (7-15Hz), theta , which is generated in the limbic system and described elsewhere, fast : 20-60 Hz, ultra-fast : 100-600 Hz. The fast and ultra-fast activities may be present in various states of vigilance including sleep and frequently coexist with slower rhythms (e.g., fast gamma oscillations may be found during depolarized phases of slow sleep oscillations). Spontaneous brain rhythms during different states of vigilance may lead to increased responsiveness and plastic changes in the strength of connections among neurons, thus affecting information flow in the thalamocortical system. -
Mechanisms of Gamma Oscillations
NE35CH10-Buzsaki ARI 22 May 2012 13:18 Mechanisms of Gamma Oscillations Gyorgy¨ Buzsaki´ 1,2 and Xiao-Jing Wang3 1Center for Molecular and Behavioral Neuroscience, Rutgers, The State University of New Jersey, Newark, New Jersey 07102 2The Neuroscience Institute, New York University, School of Medicine, New York, NY 10016; email: [email protected] 3Department of Neurobiology and Kavli Institute of Neuroscience, Yale University School of Medicine, New Haven, Connecticut 06520; email: [email protected] Annu. Rev. Neurosci. 2012. 35:203–25 Keywords First published online as a Review in Advance on inhibitory interneurons, interneuronal network, excitatory-inhibitory March 20, 2012 loop, spike timing, dynamical cell assembly, irregular spiking, The Annual Review of Neuroscience is online at cross-frequency coupling, long-distance communication neuro.annualreviews.org This article’s doi: Abstract 10.1146/annurev-neuro-062111-150444 Gamma rhythms are commonly observed in many brain regions during Copyright c 2012 by Annual Reviews. both waking and sleep states, yet their functions and mechanisms remain All rights reserved a matter of debate. Here we review the cellular and synaptic mechanisms 0147-006X/12/0721-0203$20.00 underlying gamma oscillations and outline empirical questions and controversial conceptual issues. Our main points are as follows: First, gamma-band rhythmogenesis is inextricably tied to perisomatic inhibi- by New York University - Bobst Library on 03/16/13. For personal use only. tion. Second, gamma oscillations are short-lived and typically emerge Annu. Rev. Neurosci. 2012.35:203-225. Downloaded from www.annualreviews.org from the coordinated interaction of excitation and inhibition, which can be detected as local field potentials. -
Modeling Cognitive Response to Wireless Emergency Alerts to Inform Emergency Response Interventions Final Report March 2016 PNNL-25257
Modeling Cognitive Response to Wireless Emergency Alerts to Inform Emergency Response Interventions Final Report March 2016 PNNL-25257 ii Courtney D. Corley [PI] Nathan O. Hodas Ryan Butner Joshua J. Harrison MODELING COGNITIVE Chris Berka [at ABM] RESPONSE TO WIRELESS EMERGENCY ALERTS TO INFORM EMERGENCY RESPONSE INTERVENTIONS PNNL-25257 Prepared for U.S. Department of Homeland Security Science and Technology Directorate, Support to the Homeland Security Enterprise and First Responders Group i ACKNOWLEDGMENTS Funding for this report was provided to Pacific Northwest National Laboratory by the U.S. Department of Homeland Security Science and Technology Directorate, Homeland Security Enterprise and First Responders Group. For more information about this document, contact: Pacific Northwest National Laboratory 902 Battelle Boulevard Richland, Washington 99354 Tel: 1-888-375-PNNL (7665) www.pnnl.gov Contents EXECUTIVE SUMMARY ......................................................................................................................................... 6 1.0 INTRODUCTION ......................................................................................................................................... 10 1.1 OVERVIEW OF PROJECT ................................................................................................................................. 10 1.2 BACKGROUND ON RISK COMMUNICATION ................................................................................................... 11 1.2.1 UNDERSTANDING RISK PERCEPTION -
Generation and Propagation of Slow Waves, Role in Long- Term Plasticity and Gating
SYLVAIN CHAUVETTE SLOW-WAVE SLEEP: GENERATION AND PROPAGATION OF SLOW WAVES, ROLE IN LONG- TERM PLASTICITY AND GATING Thèse présentée à la Faculté des études supérieures et postdoctorales de l’Université Laval dans le cadre du programme de doctorat en Neurobiologie pour l’obtention du grade de Philosophiae Doctor (Ph.D.) DÉPARTEMENT DE PSYCHIATRIE ET DE NEUROSCIENCES FACULTÉ DE MÉDECINE UNIVERSITÉ LAVAL QUÉBEC 2013 © Sylvain Chauvette, 2013 Résumé Le sommeil est connu pour réguler plusieurs fonctions importantes pour le cerveau et parmi celles-ci, il y a le blocage de l’information sensorielle par le thalamus et l’amélioration de la consolidation de la mémoire. Le sommeil à ondes lentes, en particulier, est considéré être critique pour ces deux processus. Cependant, leurs mécanismes physiologiques sont inconnus. Aussi, la marque électrophysiologique distinctive du sommeil à ondes lentes est la présence d’ondes lentes de grande amplitude dans le potentiel de champ cortical et l’alternance entre des périodes d’activités synaptiques intenses pendant lesquelles les neurones corticaux sont dépolarisés et déchargent plusieurs potentiels d’action et des périodes silencieuses pendant lesquelles aucune décharge ne survient, les neurones corticaux sont hyperpolarisés et très peu d’activités synaptiques sont observées. Tout d'abord, afin de mieux comprendre les études présentées dans ce manuscrit, une introduction générale couvrant l'architecture du système thalamocortical et ses fonctions est présentée. Celle-ci comprend une description des états de vigilance, suivie d'une description des rythmes présents dans le système thalamocortical au cours du sommeil à ondes lentes, puis par une description des différents mécanismes de plasticité synaptique, et enfin, deux hypothèses sur la façon dont le sommeil peut affecter la consolidation de la mémoire sont présentées. -
A Multi-Sensor Platform for Microcurrent Skin Stimulation During Slow Wave Sleep
University of Windsor Scholarship at UWindsor Electronic Theses and Dissertations Theses, Dissertations, and Major Papers 10-5-2017 A Multi-Sensor Platform for Microcurrent Skin Stimulation during Slow Wave Sleep Francia Dauz University of Windsor Follow this and additional works at: https://scholar.uwindsor.ca/etd Recommended Citation Dauz, Francia, "A Multi-Sensor Platform for Microcurrent Skin Stimulation during Slow Wave Sleep" (2017). Electronic Theses and Dissertations. 7246. https://scholar.uwindsor.ca/etd/7246 This online database contains the full-text of PhD dissertations and Masters’ theses of University of Windsor students from 1954 forward. These documents are made available for personal study and research purposes only, in accordance with the Canadian Copyright Act and the Creative Commons license—CC BY-NC-ND (Attribution, Non-Commercial, No Derivative Works). Under this license, works must always be attributed to the copyright holder (original author), cannot be used for any commercial purposes, and may not be altered. Any other use would require the permission of the copyright holder. Students may inquire about withdrawing their dissertation and/or thesis from this database. For additional inquiries, please contact the repository administrator via email ([email protected]) or by telephone at 519-253-3000ext. 3208. A Multi-Sensor Platform for Microcurrent Skin Stimulation during Slow Wave Sleep By Francia Tephanie Dauz A Thesis Submitted to the Faculty of Graduate Studies through the Department of Electrical and Computer Engineering in Partial Fulfillment of the Requirements for the Degree of Master of Applied Science at the University of Windsor Windsor, Ontario, Canada 2017 ©2017 Francia Tephanie Dauz A Multi-Sensor Platform for Microcurrent Skin Stimulation during Slow Wave Sleep By Francia Tephanie Dauz Approved By: W. -
Learning, Memory, Cognition – 1
187 Learning, Memory, Cognition – 1 P350 or two non-interfering (A–B, C–D) lists of unrelated word-pair Dream pictures, neuroholography and the laws of physics associates, to either a criterion of 90% or 60% correct. Memory I. BO´ KKON performance was tested in four groups that slept after learning and in Department of Genetics, Cell and Immunobiology, Semmelweis eight wake-control conditions (each 10 subjects of which 5 were University, Budapest, Hungary male). According to the laws of physics, electrical signals generate visible Results: For the interfering lists the data showed a release from the pictures if electrical signals are converted to electromagnetic waves retroactive interference (i.e. interference from newer information on (EMW) of the visible range (light photons of wavelengths between something learned before) that is induced by this type of learning, after 350–700 nm). During dreams our eyes are closed, so the brain is sleep (P>0.45 for memory performance on the two lists). In all the isolated from visible EMWs of the surroundings, yet we can see wake control conditions memory performance was better for the visible dream pictures. It follows from the foregoing that electrical second list than for the first one (P<0.05). For non-interfering lists signals of the brain processes can generate visible pictures of dreams memory performance was comparable for both lists in all groups if and only if electrical signals are converted to weak, EMWs of the (P>0.80). Here, enhanced memory performance for both lists was visible range (biophotons) in the brain. Nobody can explain origin of found after sleep compared to the wake control conditions but only visible dream pictures if only the laws of physics are being taken into when the lists were learned to a criterion of 60% (P<0.05 for all consideration! The homeotherm state and REM phases of sleep are relevant comparisons). -
Gamma Wave Oscillation and Synchronized Neural Signaling
_`{|}~~{}¥M{{ $% &!#'# !## '_{|}~ ## ## }¦{| ` R post hoc `~~ }{ } { Q R ~ #` |}}{ ¥ ` ||| ¦ |}}{ 8th FAOPS Congress, November 22-25, 2015, Bangkok, Thailand S-18 J Physiol Sci (2015) 65 Suppl 2: S-17 – S-22 hippocampal fibers reported to project to the lateral Electrodes were implanted according to the procedures hypothalamus [9]. described by Cheha et al. [13]. Briefly, animals were In reticulo-hypothalamic systems, a stimulation of the anesthetized with a cocktail of ketamine and xylazine via lateral hypothalamus was reported to affect the intramuscular injection and positioned in a stereotaxic hippocampal theta oscillation [10]. Electrolytic lesion of apparatus. Lidocaine was applied under the dorsal scalp the lateral hypothalamus activated aphagic mechanisms as a local anesthesia to make midline incision. Therefore, including the absence of normal atropine-resistant EEG intracranial electrodes were implanted on the left side of activity in the hippocampus [11]. Changes in the the brain to the lateral hypothalamus (anteroposterior hippocampal brain oscillation were also observed in (AP) to bregma -1.5 mm, mediolateral (ML) to bregma response to stimulation or blockade of the lateral -1 mm, dorsoventral (DV) to bregma 5.2 mm), dorsal hypothalamus [12]. These findings appeared to confirm hippocampus CA1 (AP -2.5 mm, ML -1.5 mm, DV 1.5 the connections between brain areas that some of them mm) and cerebellum (AP -6.5 mm, Midline, DV 2 mm as might function in association with feeding behaviors. a reference) according to mouse brain atlas [14]. However, the relationship between the lateral Thereafter, electrodes were connected with the skull by hypothalamus and the hippocampus in food intake using dental cement.