Exploring the Validity of the Glidewell- Lloyd Extension of Clar's Π-Sextet Rule. Assessment from Polycyclic Conjugated Hydr
Total Page:16
File Type:pdf, Size:1020Kb
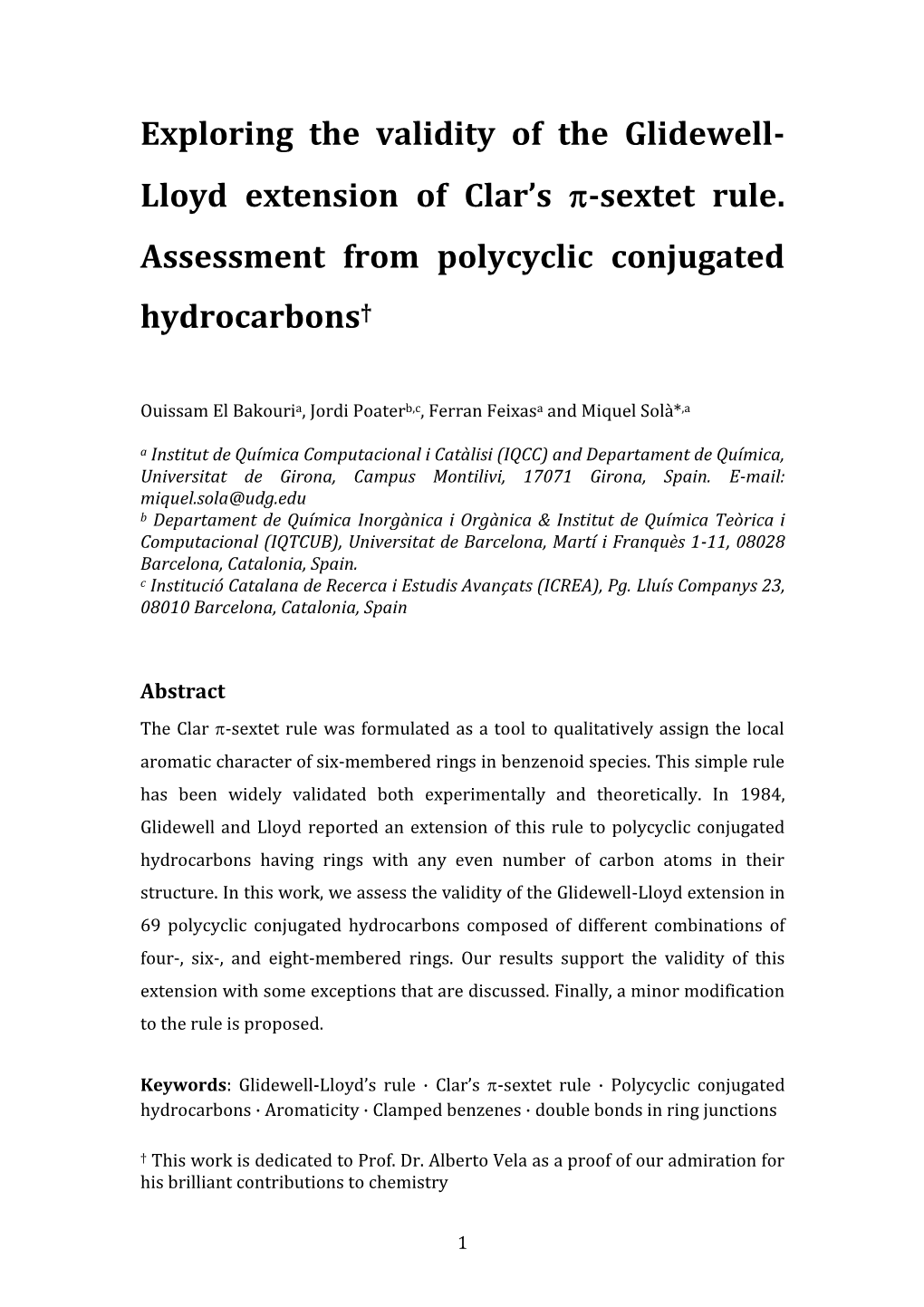
Load more
Recommended publications
-
Petrochemicals from Oil Sands
PETROCHEMICALS FROM OIL SANDS for ALBERTA ENERGY RESEARCH INSTITUTE NOVA CHEMICALS LTD. SHELL CHEMICALS CANADA SUNCOR ENERGY INC. by T. J. McCann and Associates Ltd. with Lenef Consulting (1994) Limited KemeX Engineering Services Ltd. SFA Pacific, Inc. Sigurdson & Associates July 2002 DISCLAIMER Except as specifically noted the calculations, conclusions and recommendations of this report are the sole responsibility of T. J. McCann and Associates Ltd. Advice, calculations and other assistance of Lenef Consulting (1994) Limited, KemeX Engineering Services Ltd., SFA Pacific, Inc. and Sigurdson & Associates has been incorporated herein as considered appropriate. The data, conclusions and recommendations may not concur with the opinions and/or policies of any of the sponsoring organizations. It is to be noted that the underlying data and assumptions in this report are often forecasts and/or estimates by the team and no guarantees are provided as to qualities of data or recommendations. Notices • This report was prepared for the listed sponsors, who retain the sole rights to its distribution. However, as most assumptions drew on publicly available data/information, no specific proprietary technical rights of the sponsors are inherent in this report. • Whenever specific licensors and/or processes are noted these are for illustration only, with no implied recommendations. - i – TABLE OF CONTENTS PAGE DISCLAIMER...........................................................................................................................................................................................i -
Aromatic Or Antiaromatic?
10322 J. Am. Chem. Soc. 2001, 123, 10322-10328 Butalene and Related Compounds: Aromatic or Antiaromatic? Philip M. Warner* and Graham B. Jones* Contribution from the Department of Chemistry, Northeastern UniVersity, Boston, Massachusetts 02115 ReceiVed May 7, 2001 Abstract: Density functional theory (DFT) has been used to study the first three members of the condensed cyclobutadienoid series, butalene (3), bicyclobutadienylene (12), and dicyclobutenobutalene (20). The first is planar and is judged “aromatic” by comparisons with suitable models using both energetic and magnetic criteria. The second is nonplanar, and not aromatic, but not so antiaromatic as cyclobutadiene (11). The third is slightly more antiaromatic and best viewed as a butalene fused to two cyclobutadiene rings; its properties are the sum of aromatic and antiaromatic components, like benzocyclobutadiene. Ring-opening transition states for both 3 and 12 have been located, and these are conrotatorily twisted. The ring-opening barrier for 12 is more than twice that for 3. Ring-opening of 20 involves ring inversion as the only barrier. Introduction The Bergman rearrangement of enediynes to p-benzynes (e.g., 1 to 2) continues to receive experimental and theoretical attention.1 The possibility that butalene (3), a “valence isomer” of 2, could have an independent existence was recognized over 25 years ago, when it was introduced as a theoretical species less stable than 2 and insulated from conversion to 2 by a 4.6 kcal/mol barrier (MINDO 3).2,3 The Breslow lab has reported experimental results implicating 3 as an intermediate.4,5 Briefly summarized, chloro-Dewar benzene 4 was treated with strong base to afford, in the presence of diphenylisobenzofuran, 10- 15% of amino-Dewar benzene adduct 6. -
Electronic Structure, Chemical Bonding, and Electronic Delocalization of Organic and Inorganic Systems with Three-Dimensional Or Excited State Aromaticity
ELECTRONIC STRUCTURE, CHEMICAL BONDING, AND ELECTRONIC DELOCALIZATION OF ORGANIC AND INORGANIC SYSTEMS WITH THREE-DIMENSIONAL OR EXCITED STATE AROMATICITY Ouissam El Bakouri El Farri Per citar o enllaçar aquest document: Para citar o enlazar este documento: Use this url to cite or link to this publication: http://hdl.handle.net/10803/565444 ADVERTIMENT. L'accés als continguts d'aquesta tesi doctoral i la seva utilització ha de respectar els drets de la persona autora. Pot ser utilitzada per a consulta o estudi personal, així com en activitats o materials d'investigació i docència en els termes establerts a l'art. 32 del Text Refós de la Llei de Propietat Intel·lectual (RDL 1/1996). Per altres utilitzacions es requereix l'autorització prèvia i expressa de la persona autora. En qualsevol cas, en la utilització dels seus continguts caldrà indicar de forma clara el nom i cognoms de la persona autora i el títol de la tesi doctoral. No s'autoritza la seva reproducció o altres formes d'explotació efectuades amb finalitats de lucre ni la seva comunicació pública des d'un lloc aliè al servei TDX. Tampoc s'autoritza la presentació del seu contingut en una finestra o marc aliè a TDX (framing). Aquesta reserva de drets afecta tant als continguts de la tesi com als seus resums i índexs. ADVERTENCIA. El acceso a los contenidos de esta tesis doctoral y su utilización debe respetar los derechos de la persona autora. Puede ser utilizada para consulta o estudio personal, así como en actividades o materiales de investigación y docencia en los términos establecidos en el art. -
The Thermal Transformation Mechanism of Chlorinated Paraffins: an Experimental and Density Functional Theory Study
JOURNAL OF ENVIRONMENTAL SCIENCES 75 (2019) 378– 387 Available online at www.sciencedirect.com ScienceDirect www.elsevier.com/locate/jes The thermal transformation mechanism of chlorinated paraffins: An experimental and density functional theory study Shanzhi Xin1,2, Wei Gao1,4, Dandan Cao1, Kun Lv1,6, Yaquan Liu5, Chunyan Zhao5,⁎, Yawei Wang1,3,4,⁎, Guibin Jiang1 1. State Key Laboratory of Environmental Chemistry and Ecotoxicology, Research Center for Eco-Environmental Sciences, Chinese Academy of Sciences, Beijing 100085, China 2. Hubei Key Laboratory of Industrial Fume and Dust Pollution Control, Jianghan University, Wuhan 430056, China 3. Institute of Environment and Health, Jianghan University, Wuhan 430056, China 4. University of Chinese Academy of Sciences, Beijing 100049, China 5. School of Pharmacy, Lanzhou University, Lanzhou 730000, China 6. Environment Research Institute, Shandong University, Jinan 250100, China ARTICLE INFO ABSTRACT Article history: The increasing production and usage of chlorinated paraffins (CPs) correspondently increase the Received 12 April 2018 amount of CPs that experience thermal processes. Our previous study revealed that a significant Revised 24 May 2018 amount of medium-chain chlorinated paraffins (MCCPs), short-chain chlorinated paraffins Accepted 25 May 2018 (SCCPs) as well as aromatic and chlorinated polycyclic aromatic hydrocarbons (Cl-PAHs) were Available online 8 June 2018 formed synergistically during the thermal decomposition of CP-52 (a class of CP products). However, the transformation mechanisms of CP-52 to these compounds are still not very clear. Keywords: This article presents a mechanistic analysis on the decomposition of CP-52 experimentally and Decomposition mechanism theoretically. It was found that CP-52 initially undergoes dehydrochlorination and carbon chain Chlorinated paraffins cleavage and it transformed into chlorinated and unsaturated hydrocarbons. -
Umted States Patent [19] [11] Patent Number: 4,902,539
Umted. States Patent [19] [11] Patent Number: 4,902,539 Jackson [45] Date of Patent: Feb. 20, 1990 [54] FUEL-OIHDANT NIIXTURE‘ FOR 3,910,494 10/1975 Melton, J1‘. ......................... .. 239/13 DETONAHON GUN FLAME'PLAT‘NG 1'83’??? 111171333 ‘1211' " 333%? , , verev e - n .1 [75] Inventor: John E. Jackson, Brownsburg, Ind. 4,215,819 8/1980 Garda et a1. 239/81 . 4,231,518 11/1980 Zverev et a1. .. .. 239/81 [73] Asslgnee’ Um“ ca'b‘de C°"P°“m°“' 4,258,091 3/1981 Dudko et a1. .. 427/422 Danbury, Cmm- 4,279,383 7/1981 Zverev et a1. .. 239/61 _ 4,319,715 3/1982 Garda et a1. .. 239/81 [21] Appl' N°f' 146’723 4,469,772 9/1984 Barton et 111. ..... .. 430/163 [22] Filed: Feb. 4, 1988 4,621,017 11/1986 Chandler et a1. 427/430.1 X 4,669,658 6/ 1987 Nevgod et a1. .. 239/81 - 4,687,673 8/ 1987 Lindblom ..... .. 427/423 X Ram“! U‘S‘ Amman Dm 4,731,253 3/1988 1511116111 .......................... .. 427/423 x [ 63 1 abaldo‘ffefnC0 tin ti -'m -Pam fSe ' .N° . 110,841, Oct. 21, 1987, OTHER PUBLICATIONS 4 Deposition of Coatings by Detonation Methods, A. I. [51] Int. Cl. ............................................. .. 13051) 1/10 _ _ , [52] US. 01. ........ ..... ..427/423;149/109.2; Zverev 6* 91- Lemngrad' Swdestwyemye Pubhshers, 239/13; 252/372; 89/7 1979 ‘pp. 31-32 and pp. 165-166. I [58] Field of Search ................ .. 427/422, 423; 239/81, ' C°atm8§ Based On Refractory Compounds Apphed by 239/13; 252/372; 149/1092; 89/7 1361291131101! V. -
Modern Valence-Bond Descriptions of Polycyclic Fused Aromatic Compounds Involving Cyclopropenyl Rings
View metadata, citation and similar papers at core.ac.uk brought to you by CORE provided by University of Liverpool Repository Modern Valence-Bond Descriptions of Polycyclic Fused Aromatic Compounds Involving Cyclopropenyl Rings a, b, Peter B. Karadakov and David L. Cooper aDepartment of Chemistry, University of York, Heslington, York, YO10 5DD, U.K. b Department of Chemistry, University of Liverpool, Liverpool L69 7ZD, U.K. Abstract The feasibilities and electronic structures of five ten--electron fused conjugated molecules involv- ing cyclopropenyl rings are explored using second-order Møller-Plesset perturbation theory (MP2), spin-coupled (SC) and complete-active-space self-consistent-field (CASSCF) wavefunctions, in the cc-pVTZ basis. All five fused conjugated molecules are predicted to have rigid planar ground state geometries of C2v or D2h symmetry and large dipole moments (if not of D2h symmetry). The com- pact ground state SC(10) wavefunctions with ten active orbitals for these molecules are found to be of comparable quality to the respective CASSCF(10,10) constructions, but much easier to interpret. The analyses of the ground state SC(10) wavefunctions for all five fused conjugated molecules re- veal resonance patterns which indicate that all of these molecules are aromatic in their electronic ground states; on the other hand, the SC(10) approximations to the first singlet electronic excited states are found to exhibit “antiresonance” which suggests that each of the five molecules switches from aromatic to antiaromatic upon vertical excitation from the ground state to its first singlet excited state. Ring strain prevents the formation of a fused structure involving three cyclopropenyl rings and a cycloheptatrienyl ring; the alternative stable dehydro compound which resembles m-benzyne is shown, using a SC(12) wavefunction, to involve a weak bond between the dehydro centres. -
Quantitative Studies on Aromaticity and Antiaromaticity
QUANTITATIVE STUDIES ON AROMATICITY AND ANTIAROMATICITY RONALD BRESLOW Department of Chemistry, Columbia University, New York, N.Y. 10027, USA ABSTRACT The properties of the simplest aromatic compound, are fully consistent with expectations. Spectroscopic studies show that it is a symmetric cation with approximately the stability expected from simple quantum mechanical con- siderations. Various studies on cyclopropenyl anions reveal that they are undoubtedly resonance destabilized. Thus, the kinetic studies which have been done on rates of hydrogen exchange in this system can be shown to be consistent only with electronic destabilization in the antiaromatic cyclopropenyl anion. Direct thermodynamic determinations of the stability of cyclopropenyl anions can also be performed using a novel electrochemical procedure, and using this method very high PKaS are found for some of these anions, indicating great stability. Resonance destabilization in cyclobutadiene can also be demon- strated by examining the basicity of a cyclobutadienocyclopentadiene anion and also by electrochemical studies on a fused naphthalenecyclobutadiene derivative. 1-Chloro-bicyclo{2,2,O]hexadiene has been prepared. On treatment with strong base it apparently eliminates HC1 to generate transient butalene (cyclobutadienocyclobutadiene) which is then trapped by the medium. Since the first synthesis of a cyclopropenyl cation, the triphenyl derivative synthesized in 19671, work in our laboratory and in a number of others has been devoted to a study o this simplest aromatic system. Of course, the description of the cyclopropenyl cation ring as 'aromatic' involves some assumptions about a definition of the term aromaticity, and I would like to focus first on this problem. There are a number of things which are special about the compounds which have been called aromatic, but the one which is certainly at the root of most of their unusual properties concerns their energies. -
Modern Valence-Bond Descriptions of Polycyclic Fused Aromatic Compounds Involving Cyclopropenyl Rings
This is a repository copy of Modern Valence-Bond Descriptions of Polycyclic Fused Aromatic Compounds Involving Cyclopropenyl Rings. White Rose Research Online URL for this paper: https://eprints.whiterose.ac.uk/112130/ Version: Accepted Version Article: Karadakov, Peter Borislavov orcid.org/0000-0002-2673-6804 and Cooper, David (2017) Modern Valence-Bond Descriptions of Polycyclic Fused Aromatic Compounds Involving Cyclopropenyl Rings. Computational and Theoretical Chemistry. ISSN 2210-271X https://doi.org/10.1016/j.comptc.2017.01.003 Reuse This article is distributed under the terms of the Creative Commons Attribution-NonCommercial-NoDerivs (CC BY-NC-ND) licence. This licence only allows you to download this work and share it with others as long as you credit the authors, but you can’t change the article in any way or use it commercially. More information and the full terms of the licence here: https://creativecommons.org/licenses/ Takedown If you consider content in White Rose Research Online to be in breach of UK law, please notify us by emailing [email protected] including the URL of the record and the reason for the withdrawal request. [email protected] https://eprints.whiterose.ac.uk/ Modern Valence-Bond Descriptions of Polycyclic Fused Aromatic Compounds Involving Cyclopropenyl Rings Peter B. Karadakova, and David L. Cooperb, aDepartment of Chemistry, University of York, Heslington, York, YO10 5DD, U.K. bDepartment of Chemistry, University of Liverpool, Liverpool L69 7ZD, U.K. Abstract The feasibilities and electronic structures of five ten--electron fused conjugated molecules involv- ing cyclopropenyl rings are explored using second-order Møller-Plesset perturbation theory (MP2), spin-coupled (SC) and complete-active-space self-consistent-field (CASSCF) wavefunctions, in the cc-pVTZ basis. -
(12) United States Patent (10) Patent No.: US 6,168,885 B1 Narang Et Al
USOO6168885B1 (12) United States Patent (10) Patent No.: US 6,168,885 B1 Narang et al. (45) Date of Patent: Jan. 2, 2001 (54) FABRICATION OF ELECTRODES AND 10-172615 - 6/1998 (JP). DEVICES CONTAINING ELECTRODES * cited by examiner (75) Inventors: Subhash C. Narang, Palo Alto; Susanna Ventura, Los Altos; Philip Primary Examiner Maria Nuzzolillo Cox, San Jose, all of CA (US) Assistant Examiner Susy Tsang (74) Attorney, Agent, or Firm-Fish & Associates, LLP, (73) ASSignee: SRI International, Menlo Park, CA Robert Fish (US) (57) ABSTRACT (*) Notice: Under 35 U.S.C. 154(b), the term of this Electrodes are manufactured using a fire-retardant Solvent patent shall be extended for 0 days. and a polymerizable monomer. Electrodes according to the present invention are contemplated to find applicability in (21) Appl. No.: 09/226,558 Substantially any electrode containing device, including batteries. A preferred class of fire-retardant Solvents includes (22) Filed: Jan. 5, 1999 Solvents that generate a fire-retardant gas upon decomposi Related U.S. Application Data tion. One Subclass includes compositions that produce car bon dioxide upon decomposition. Other Subclasses include (63) Continuation-in-part of application No. 09/149,880, filed on Species that generate non-CO2 gases upon decomposition, Sep. 8, 1998. such as CO, SO, SO, NO, NO, NO, or N. A second (60) Provisional application No. 60/097,706, filed on Nov. 29, preferred class of fire-retardant Solvents include Solvents 1998. that are fire-retardant without generating a fire-retardant gas (51) Int. Cl. .................................................... HO1 M 4/60 upon decomposition, and are electrochemically inactive. -
Notes on Molecular Orbital Calculations
Molecular Orbital Calculations BOOKS BY JOHIC' D. ROBERTS Basic Organic Chemistry, Part I Nuclear Magnetic Resonance, Applications to Organic Chemistry An Introduction to the Analysis of Spin-Spin Splitting in High-Re solution Nuclear Magnetic Resonance Spectra Notes on Molecular Orbital ~alculations John D. Roberts Professor of Organic Chemistry California Institute of Technology Illustrated by the Author 1961 THE BENJAMINICUMMIMGS PUBLlSHING COMPANY, INC. ADVANCED BOOK PROGRAM Reading, Massachusetts London . Amsterdam Don Mills, Ontario . Sydney . Tokyo NOTES ON MOLECULAR ORBITAL CALCULATIONS First printing, 1961 Second printing, with corrections, 1962 Third printing-Twelfth printing, 1963-1 970 Thirteenth printing, 19 74 Fourteenth printing, 1978 The publisher wishes to express his appreciation to Dr. Roberts, who, in addition to writing the manuscript, prepared all the illustrations. The author was also responsible for the editing and composition; final pages, ready for the camera, were typed at the California Institute of Technology under Dr. Roberts' supervision. International Standard Book Number: 0-8053-8301-8(paperback) Library of Congress Catalog Card Number: 61-1 81 59 Copyright @ 1961 by W. A. Benjamin, Inc. All rights reserved. No part of this publication may be reproduced, stored in a retrieval system, or transmitted, in any form or by any means, electronic, mechanical, photocopying, recording, or otherwise, without the prior written permission of the publisher, W. A. Benjamin, Inc., Advanced Book Program, Reading, Massachusetts, 01867, U.S.A. Manufactured in the United States of America. ISBN 080538301-8 ABCDEFGHIJ-AL-78 Certificate of Recordation THIS IS TO CERTIFY THAT THE ATTACHED DOCU- MENT WAS RECORDED IN THE COPYRIGHT OFFICE ON THE DATE AND IN THE PLACE SHOWN BELOW. -
Steam, the Necessity of the Safety Device Became Evident
Introduction to Safety Valves Introduction As soon as mankind was able to boil water to create steam, the necessity of the safety device became evident. As long as 2000 years ago, the Chinese were using cauldrons with hinged lids to allow (relatively) safer production of steam. At the beginning of the 14th century, chemists used conical plugs and later, compressed springs to act as safety devices on pressurised vessels. Early in the 19th century, boiler explosions on ships and locomotives frequently resulted from faulty safety devices, which led to the development of the first safety relief valves. In 1848, Charles Retchie invented the accumulation chamber, which increases the compression surface within the safety valve allowing it to open rapidly within a narrow overpressure margin. Today, most steam users are compelled by local health and safety regulations to ensure that their plant and processes incorporate safety devices and precautions, which ensure that dangerous conditions are prevented. The primary function of a safety valve is therefore to protect life and property. The principle type of device used to prevent overpressure in plant is the safety or safety relief valve. The safety valve operates by releasing a volume of fluid from within the plant when a predetermined maximum pressure is reached, thereby reducing the excess pressure in a safe manner. As the safety valve may be the only remaining device to prevent catastrophic failure under overpressure conditions, it is important that any such device is capable of operating at all times and under all possible conditions. Safety valves should be installed wherever the maximum allowable working pressure (MAWP) of a system or pressure-containing vessel is likely to be exceeded. -
Robert G. Bergman's Publications
Publications 543. Mugridge, J.S.; Bergman, R.G.; Raymond, K.N. “Equilibrium Isotope Effects on Noncovalent Interactions in a Supramolecular Host−Guest System” J. Am. Chem. Soc. 2012, 134, 2057-2066. 542. Tauchert, M.E.; Incarvito, C.D.; Rheingold, A.L.; Bergman, R.G.; Ellman, J.A. “Mechanism of the Rhodium(III)-Catalyzed Arylation of Imines via C–H Bond Functionalization: Inhibition by Substrate” J. Am. Chem. Soc. 2012, 134, 1482-1485. 541. Gianetti, T.L; Tomson, N.C.; Arnold, J.; Bergman, R.G. “Z-selective Catalytic Internal- Alkyne Semi-Hydrogenation under H2:CO Mixtures by a Niobium(III) Imido Complex” J. Am. Chem. Soc. 2011, 133, 14904-14907. 540. Tomson, N.C.; Crimmin, M.R.; Petrenko, T.; Rosebrugh, L.E.; Sproules, S.; Boyd, W.C.; Bergman, R.G.; DeBeer, S.; Toste, F.D.; Wieghardt, K. “A Step beyond the Feltham_Enemark Notation: Spectroscopic and Correlated ab Initio Computational Support for an Antiferromagnetically Coupled M(II) ̶ (NO)¯ Description of Tp*M(NO)(M = Co, Ni)” J. Am. Chem. Soc. 2011, 133, 18785-18801. 539. Crimmin, M.R.; Rosebrugh, L.E.; Tomson, N.C., Weyhermüller, T.; Bergman, R.G.; Toste, F.D.; Wieghardt, K. “[(TMEDA)Co(NO)2][BPh4]: A Versatile Synthetic Entry Point to Four and Five Coordinate {Co(NO)2}10 Complexes” J. Organometallic Chem. 2011, 696, 3974-3981. 538. Brown, C.J.; Miller, G.M.; Johnson, M.W.; Bergman, R.G.; Raymond, K.N. “High- Turnover Supramolecular Catalysis by a Protected Ruthenium(II) Complex in Aqueous Solution” J. Am. Chem. Soc. 2011, 133, 11964-11966. 537. Zhao, C.; Bergman, R.G.; Toste, F.D.