Synthetic Methodology Towards ADP-Ribosylation Related Molecular Tools
Total Page:16
File Type:pdf, Size:1020Kb
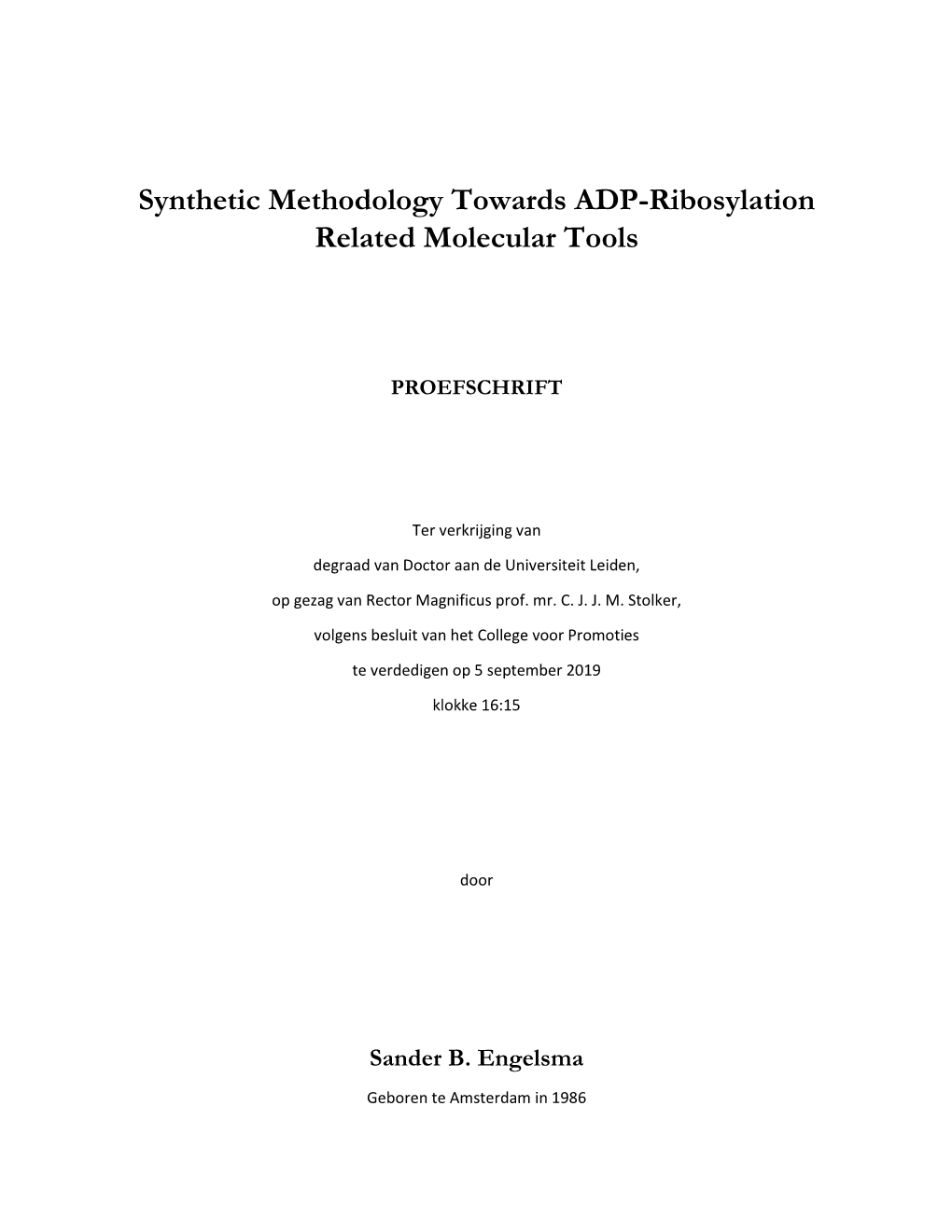
Load more
Recommended publications
-
Chronic Pelvic Pain M
Guidelines on Chronic Pelvic Pain M. Fall (chair), A.P. Baranowski, S. Elneil, D. Engeler, J. Hughes, E.J. Messelink, F. Oberpenning, A.C. de C. Williams © European Association of Urology 2008 TABLE OF CONTENTS PAGE 1. INTRODUCTION 5 1.1 The guideline 5 1.1.1 Publication history 5 1.2 Level of evidence and grade recommendations 5 1.3 References 6 1.4 Definition of pain (World Health Organization [WHO]) 6 1.4.1 Innervation of the urogenital system 7 1.4.2 References 8 1.5 Pain evaluation and measurement 8 1.5.1 Pain evaluation 8 1.5.2 Pain measurement 8 1.5.3 References 9 2. CHRONIC PELVIC PAIN 9 2.1 Background 9 2.1.1 Introduction to urogenital pain syndromes 9 2.2 Definitions of chronic pelvic pain and terminology (Table 4) 11 2.3 Classification of chronic pelvic pain syndromes 12 Table 3: EAU classification of chronic urogenital pain syndromes (page 10) Table 4: Definitions of chronic pain terminology (page 11) Table 5: ESSIC classification of types of bladder pain syndrome according to the results of cystoscopy with hydrodistension and of biopsies (page 13) 2.4 References 13 2.5 An algorithm for chronic pelvic pain diagnosis and treatment 13 2.5.1 How to use the algorithm 13 2.6 Prostate pain syndrome (PPS) 15 2.6.1 Introduction 16 2.6.2 Definition 16 2.6.3 Pathogenesis 16 2.6.4 Diagnosis 17 2.6.5 Treatment 17 2.6.5.1 Alpha-blockers 17 2.6.5.2 Antibiotic therapy 17 2.6.5.3 Non-steroidal anti-inflammatory drugs (NSAIDs) 17 2.6.5.4 Corticosteroids 17 2.6.5.5 Opioids 17 2.6.5.6 5-alpha-reductase inhibitors 18 2.6.5.7 Allopurinol 18 2.6.5.8 -
G Protein-Coupled Receptors As Therapeutic Targets for Multiple Sclerosis
npg GPCRs as therapeutic targets for MS Cell Research (2012) 22:1108-1128. 1108 © 2012 IBCB, SIBS, CAS All rights reserved 1001-0602/12 $ 32.00 npg REVIEW www.nature.com/cr G protein-coupled receptors as therapeutic targets for multiple sclerosis Changsheng Du1, Xin Xie1, 2 1Laboratory of Receptor-Based BioMedicine, Shanghai Key Laboratory of Signaling and Disease Research, School of Life Sci- ences and Technology, Tongji University, Shanghai 200092, China; 2State Key Laboratory of Drug Research, the National Center for Drug Screening, Shanghai Institute of Materia Medica, Chinese Academy of Sciences, 189 Guo Shou Jing Road, Pudong New District, Shanghai 201203, China G protein-coupled receptors (GPCRs) mediate most of our physiological responses to hormones, neurotransmit- ters and environmental stimulants. They are considered as the most successful therapeutic targets for a broad spec- trum of diseases. Multiple sclerosis (MS) is an inflammatory disease that is characterized by immune-mediated de- myelination and degeneration of the central nervous system (CNS). It is the leading cause of non-traumatic disability in young adults. Great progress has been made over the past few decades in understanding the pathogenesis of MS. Numerous data from animal and clinical studies indicate that many GPCRs are critically involved in various aspects of MS pathogenesis, including antigen presentation, cytokine production, T-cell differentiation, T-cell proliferation, T-cell invasion, etc. In this review, we summarize the recent findings regarding the expression or functional changes of GPCRs in MS patients or animal models, and the influences of GPCRs on disease severity upon genetic or phar- macological manipulations. -
Pepper Spray: What Do We Have to Expect?
Pepper Spray: What Do We Have to Expect? Assoc. Prof. Mehmet Akif KARAMERCAN, MD Gazi University School of Medicine Department of Emergency Medicine Presentation Plan • History • Pepper Spray • Tear Gas • Symptoms • Medical Treatment • If you are the victim ??? History • PEPPER SPRAY ▫ OC (oleoresin of capsicum) (Most Commonly Used Compound) • TEAR GAS ▫ CN (chloroacetophenone) (German scientists 1870 World War I and II) ▫ CS (orthochlorobenzalmalononitrile) (US Army adopted in 1959) ▫ CR (dibenzoxazepine) (British Ministry of Defence 1950-1960) History of Pepper Spray • Red Chili Pepper was being used for self defense in ancient India - China - Japan (Ninjas). ▫ Throw it at the faces of their enemies, opponents, or intruders. • Japan Tukagawa Empire police used a weapon called the "metsubishi." • Accepted as a weapon ▫ incapacitate a person temporarily. • Pepper as a weapon 14th and 15th century for slavery rampant and became a popular method for torturing people (criminals, slaves). History of Pepper Spray • 1980's The USA Postal Workers started using pepper sprays against dogs, bears and other pets and became a legalized non-lethal weapon ▫ Pepper spray is also known as oleoresin of capsicum (OC) spray • The FBI in 1987 endorse it as an official chemical agent and it took 4 years it could be legally accepted by law enforcement agency. Pepper Spray • The active ingredient in pepper spray is capsaicin, which is a chemical derived from the fruit of plants of chilis. • Extraction of Oleoresin Capsicum from peppers ▫ capsicum to be finely ground, capsaicin is then extracted using an organic solvent (ethanol). The solvent is then evaporated, remaining waxlike resin is the Oleoresin Capsicum • Propylene Glycol is used to suspend the OC in water, pressurized to make it aerosol in Pepper Spray. -
Toxic Materials to Cornea INTRODUCTION
International Journal of Veterinary and Animal Research Uluslararası Veteriner ve Hayvan Araştırmaları Dergisi E-ISSN: 2651-3609 2(1): 06-10, 2019 Toxic Materials to Cornea Eren Ekici1, Ender Yarsan2* 1Ankara Ulucanlar Eye Training and Research Hospital, Department of Ophtalmology, Ankara, Turkey 2Ankara University Faculty of Veterinary Medicine, Department of Pharmacology and Toxicology, Ankara, Turkey *Corresponding Author Received: February 12, 2019 E-mail:[email protected] Accepted: March 6, 2019 Abstract Every day; many chemical agents, materials or medicines whether in the pharmaceutical industry or daily life are offered for consuming for human beings. At this point, it has great importance that if the substances threaten heath or not. Because the toxicity of materials can lead to many target organ damage. The eye, together with many anatomical layers that make it up is among the target organs exposed to toxicity. In this review, we handled the classification, effects and treatment methods of toxic materials on the corneal layer of the eye. Keywords: Cornea, toxic materials, chemicals, eye INTRODUCTION and materials known to be toxic in high-risk situations (ie Toxic material is a chemical substance that breaks down aminoglycosides, some glaucoma medications, antivirals, normal physiological and biochemical mechanisms when chronic disease, dry eyes and patients on multiple topical it enters the living organism (human and warm-blooded therapies) is effective to protect from toxicity (Dart, 2003). animals) through mouth, respiration, skin, and infection, or Table 1: Classification by route of exposure and time course. causes the death of the creature in an excess amount. For Local action, immediate corneal toxicity; there are many methods of classification Examples effects based on the disease, route of exposure and duration or agent Caustic chemicals Acids and alkalis (Grant, 1986). -
Weaponizing Tear Gas: Bahrain’S Unprecedented Use of Toxic Chemical Agents Against Civilians
Physicians for Human Rights Weaponizing Tear Gas: Bahrain’s Unprecedented Use of Toxic Chemical Agents Against Civilians August 2012 physiciansforhumanrights.org About Physicians for Human Rights Physicians for Human Rights (PHR) uses medicine and science to investigate and expose human rights violations. We work to prevent rights abuses by seeking justice and holding offenders accountable. Since 1986, PHR has conducted investigations in more than 40 countries, including on: 1987 — Use of toxic chemical agents in South Korea 1988 — Iraq’s use of chemical weapons against Kurds 1988 — Use of toxic chemical agents in West Bank and the Gaza Strip 1989 — Use of chemical warfare agents in Soviet Georgia 1996 — Exhumation of mass graves in the Balkans 1996 — Critical forensic evidence of genocide in Rwanda 1999 — Drafting the UN-endorsed guidelines for documentation of torture 2004 — Documentation of the genocide in Darfur 2008 — US complicity of torture in Iraq, Afghanistan, and Guantánamo Bay 2010 — Human experimentation by CIA medical personnel on prisoners in violation of the Nuremberg Code 2011 — Violations of medical neutrality in times of armed conflict and civil unrest during the Arab Spring ... 2 Arrow Street | Suite 301 1156 15th Street, NW | Suite 1001 Cambridge, MA 02138 USA Washington, DC 20005 USA +1 617 301 4200 +1 202 728 5335 physiciansforhumanrights.org ©2012, Physicians for Human Rights. All rights reserved. ISBN: 1-879707-68-3 Library of Congress Control Number: 2012945532 Cover photo: Bahraini anti-riot police fire tear gas grenades at peaceful and unarmed civilians protesters, including a Shi’a cleric, in June 2012. http://www.youtube.com/watch?v=QxauI5hdjqk. -
Lantern Parade & Magical Evening
Greater New Lodge Community Magazine December 2014 Ashton Centre, 5 Churchill Street, Belfast BT15 2BP Tel: (028) 90742255 email: [email protected] Thousands Attend Lantern Parade & Magical Evening Section of the crowd who took part in the Lantern Parade The North Belfast Lantern Parade and Magical Festival for the parade. took place this year on Wednesday 29th & Thursday 30th The parade itself left Crumlin Road Gaol, went along October in the Waterworks Park. During the day there was Cliftonpark Avenue, down Cliftonville Road and up the Antrim a range of activities involving street theatre performances, Road into the Waterworks Park. There were approximately storytelling, drumming, music by Cool FM’s Ryan A and visual 850 children and adults taking part in this fabulous carnival art workshops. The weather was lovely which made being parade along with performers from Fire Poise, Streetwise outdoors all the more enjoyable. Circus, and Arts Ekta. Motorway Site Continued on Page 3 - Page 4 for Photos The North Belfast Lantern Parade is part of the North Belfast Community Pride Programme which is funded through OFMDFM’s Good Relations Programme and led by Ashton Inside Highlights Community Trust. This programme is delivered by New Page 3 - Christmas Party Lodge Arts and supported by a steering group of various Page 5 - Ashton Community Bursaries North Belfast based youth and community organisations. Page 6 - Tar Isteach Face Funding Crisis Other funders for this project include Belfast City Council, Page 7 - Misuse of Prescription Drugs Community Foundation Northern Ireland and Department of Page 8 - BOH Peace Building Lessons In Berlin Foreign Affairs. -
Utilization of Various Analogy of Synthetic Nanoporous Zeolites and Composite of Zeolites for Decontamination/Detoxification of CWA Simulants—An Updated Review
International Journal of Nonferrous Metallurgy, 2019, 8, 35-71 https://www.scirp.org/journal/ijnm ISSN Online: 2168-2062 ISSN Print: 2168-2054 Utilization of Various Analogy of Synthetic Nanoporous Zeolites and Composite of Zeolites for Decontamination/Detoxification of CWA Simulants—An Updated Review Neeraj Kumar*, Kautily Rao Tiwari, Km. Meenu, Arti Sharma, Adya Jain, Shikha Singh, Radha Tomar School of Studies in Chemistry, Jiwaji University, Gwalior, India How to cite this paper: Kumar, N., Tiwari, Abstract K.R., Meenu, Km., Sharma, A., Jain, A., Singh, S. and Tomar, R. (2019) Utilization In this review, we summaries the past few year work on the chemistry of of Various Analogy of Synthetic Nanopor- CWA’s and their simulants on various heterogeneous surfaces of zeolites, ous Zeolites and Composite of Zeolites for composites of zeolites and doped zeolite with transition metal oxides. This Decontamination/Detoxification of CWA Simulants—An Updated Review. Interna- review elaborates an updated literature overview on the degradation of tional Journal of Nonferrous Metallurgy, 8, CWA’s and its simulants. The data written in this review were collected from 35-71. the peer-reviewed national and international literature. https://doi.org/10.4236/ijnm.2019.84004 Keywords Received: May 4, 2019 Accepted: October 27, 2019 Zeolite, Composites, Adsorption, Decontamination, Metal Oxide, CWA, Published: October 30, 2019 Simulants Copyright © 2019 by author(s) and Scientific Research Publishing Inc. This work is licensed under the Creative Commons Attribution International 1. Introduction License (CC BY 4.0). 1.1. Zeolites http://creativecommons.org/licenses/by/4.0/ Open Access Zeolites were first of all observed in 1756 by a Swedish mineralogist, Fredish Cronstedt. -
CS Gas Exposure in a Crowded Night Club: the Consequences for an Accident and Emergency Department
56 Case reports 9 Selbst SM, De Maio JG, Boenning D. Mouth wash poison- 13 Simon HK, Cox JM, Sucov A, Linakis JG. Ethanol ing. Clin Pediatr 1985;24:162-3. clearance in intoxicated children and adolescents present- 10 Wright J. Ethanol-induced hypoglycaemia. Br J Alcohol ing to the ED. Acad Emerg Med 1994;1:520-4. Alcoholism 1979;14:174-6. 14 Gibson PJ, Cant AJ, Mant TGK. Ethanol poisoning. Acta 11 Ricci LR, Hoffman S. Ethanol induced hypoglycaemic Paediatr Scand 1985;74:977-8. J Accid Emerg Med: first published as 10.1136/emj.15.1.56 on 1 January 1998. Downloaded from coma in a child. Ann Emerg Med 1982;1 1:203-4. 15 Pollack CV, Jorden RC, Carlton FB, Baker ML. Gastric 12 Gershman H, Steeper J. Rate of clearance of ethanol from emptying in the acutely inebriated patient. J Emerg Med the blood of intoxicated patients in the emergency depart- 1992; 10: 1-5. ment. J Emerg Med 199 1;9:307-1 1. See also letters on p00 CS gas exposure in a crowded night club: the consequences for an accident and emergency department A Breakell, G G Bodiwala Abstract respiratory problems, seven required supple- A case is reported of deliberate release of mental oxygen. Two of these patients suffered CS gas (O-chlorobenzylidene malononi- from asthma. Clinically none of the patients trile) in an enclosed space and the conse- developed wheeze but one asthmatic patient quences for an accident and emergency required a nebuliser for chest tightness. One department. patient was admitted to hospital with persistent (7AccidEmergMed 1998;15:56-64) chest tightness and sore throat. -
An Important Role for N-Acylethanolamine Acid Amidase in the Complete Freund’S Adjuvant Rat Model of Arthritis S
Supplemental material to this article can be found at: http://jpet.aspetjournals.org/content/suppl/2016/01/14/jpet.115.230516.DC1 1521-0103/356/3/656–663$25.00 http://dx.doi.org/10.1124/jpet.115.230516 THE JOURNAL OF PHARMACOLOGY AND EXPERIMENTAL THERAPEUTICS J Pharmacol Exp Ther 356:656–663, March 2016 Copyright ª 2016 by The American Society for Pharmacology and Experimental Therapeutics An Important Role for N-Acylethanolamine Acid Amidase in the Complete Freund’s Adjuvant Rat Model of Arthritis s F. T. Bonezzi, O. Sasso, S. Pontis, N. Realini, E. Romeo, S. Ponzano, A. Nuzzi, A. Fiasella, F. Bertozzi, and D. Piomelli Drug Discovery and Development, Istituto Italiano di Tecnologia, Genova, Italy (F.T.B., O.S., S.P., N.R., E.R., S.P., A.N., A.F., F.B., D.P.); and Departments of Anatomy and Neurobiology, Pharmacology and Biological Chemistry, University of California, Irvine, California (D.P.) Received November 5, 2015; accepted January 12, 2016 Downloaded from ABSTRACT The endogenous lipid amides, palmitoylethanolamide (PEA) and accompanied by decreased PEA and OEA content (assessed oleoylethanolamide (OEA), exert marked antinociceptive and by liquid chromatography/mass spectrometry) and increased anti-inflammatory effects in animal models by engaging nuclear NAAA levels (assessed by Western blot and ex vivo enzyme peroxisome proliferator-activated receptor-a. PEA and OEA are activity measurements) in paw tissue. Administration of undec- produced by macrophages and other host-defense cells and are 10-ynyl-N-[(3S)-2-oxoazetidin-3-yl] carbamate (ARN14686), a jpet.aspetjournals.org deactivated by the cysteine amidase, N-acylethanolamine acid NAAA-preferring activity-based probe, revealed that NAAA was amidase (NAAA), which is highly expressed in macrophages and catalytically active in CFA-treated paws. -
Health Impacts of Chemical Irritants Used for Crowd Control: a Systematic Review of the Injuries and Deaths Caused by Tear Gas and Pepper Spray Rohini J
Haar et al. BMC Public Health (2017) 17:831 DOI 10.1186/s12889-017-4814-6 RESEARCH ARTICLE Open Access Health impacts of chemical irritants used for crowd control: a systematic review of the injuries and deaths caused by tear gas and pepper spray Rohini J. Haar1*, Vincent Iacopino2, Nikhil Ranadive3, Sheri D. Weiser4 and Madhavi Dandu4 Abstract Background: Chemical irritants used in crowd control, such as tear gases and pepper sprays, are generally considered to be safe and to cause only transient pain and lacrimation. However, there are numerous reports that use and misuse of these chemicals may cause serious injuries. We aimed to review documented injuries from chemical irritants to better understand the morbidity and mortality associated with these weapons. Methods: We conducted a systematic review using PRISMA guidelines to identify injuries, permanent disabilities, and deaths from chemical irritants worldwide between January 1, 1990 and March 15, 2015. We reviewed injuries to different body systems, injury severity, and potential risk factors for injury severity. We also assessed region, context and quality of each included article. Results: We identified 31 studies from 11 countries. These reported on 5131 people who suffered injuries, two of whom died and 58 of whom suffered permanent disabilities. Out of 9261 total injuries, 8.7% were severe and required professional medical management, while 17% were moderate and 74.3% were minor. Severe injuries occurred to all body systems, with the majority of injuries impacting the skin and eyes. Projectile munition trauma caused 231 projectile injuries, with 63 (27%) severe injuries, including major head injury and vision loss. -
TRPS and Migraine Romina Nassini*, Francesco De Cesaris, Pamela Pedretti and Pierangelo Geppetti
The Open Drug Discovery Journal, 2010, 2, 55-63 55 Open Access TRPS and Migraine Romina Nassini*, Francesco De Cesaris, Pamela Pedretti and Pierangelo Geppetti Headache Center, University Hospital Careggi and Department of Preclinical and Clinical Pharmacology, University of Florence, Viale Pieraccini 6, 50139, Florence, Italy Abstract: Migraine is a highly prevalent, disabling neurovascular disorder characterized by a combination of headache, nausea and altered sensory processing such as photophobia. Migraine has a strong genetic background but the molecular pathways that result in a migraine attack, and the role of various triggers, are poorly understood. The throbbing and pulsating pain associated with the headache phase of migraine attack implies an important role for the nociceptive activation of trigeminal intracranial afferents that contain calcitonin gene-related peptide (CGRP). Neurogenic inflammation triggered by the release of CGRP is now recognized as a significant underlying event in migraine. Indeed, CGRP receptor antagonists, the so-called “gepants”, have already proved effective in clinical trials as novel, migraine- specific drugs. An alternative therapeutic approach is the modulation of CGRP release. As potential targets, the transient receptor potential (TRP) channels expressed by a subpopulation of CGRP-containing nociceptive primary sensory neurons are gaining increasing prominence, principally because of the recent discovery of a variety of endogenous and exogenous TRP agonists known to induce migraine attack as well as their emerging role in neuropeptide release. The present review focuses on the potential role of the different TRP channels, especially TRPV1, in the migraine mechanism. Keywords: TRP channel, TRPV1, migraine, capsaicin, CGRP, neurogenic inflammation, clinical trials, heat, neuropeptides, geptins. -
Oleoylethanolamide in the Homeostatic and Non- Homeostatic Control of Eating
Oleoylethanolamide in the homeostatic and non- homeostatic control of eating A Dissertation in Fulfillment of the Requirements for the Degree of Doctor of Philosophy in Pharmacology and Toxicology Cristina Anna Gallelli Department of Physiology and Pharmacology “Vittorio Erspamer” Sapienza University of Rome Director of the Ph.D Program: Thesis Committee: Prof. Maura Palmery Prof. Paola Casolini Thesis Advisor: Prof. Grazia Graziani Prof. Silvana Gaetani Prof. Luigia Trabace ACADEMIC YEAR 2017-2018 Index 1. Chapter I: General Introduction 1.1. Obesity and eating-related disorders ………………………………………………………..2 1.1.1. The binge eating disorder (BED) …………………………………………………….3 1.2. Neurobiological mechanisms regulating energy homeostasis ………………………………4 1.2.1. Hormonal Signals …………………………………………………………………….9 1.2.2. Neuropeptidergic system …………………………………………………………...13 1.2.2.1. Oxytocinergic system ……………………………………………………….15 1.2.3. Neurotransmitter systems …………………………………………………………...17 1.3. Neurobiological mechanisms regulating stress ……………………………………………23 1.4. Neurobiological mechanisms regulating food addiction …………………………………..25 1.5. Pharmacological treatment for obesity and BED ………………………………………….27 1.6. Oleoylethanolamide and N-acylethanolamides as lipid mediators ………………………..32 1.6.1. Synthesis and metabolism …………………………………………………………..33 1.6.2. Receptors ……………………………………………………………………………35 1.6.3. OEA and the control of food intake ………………………………………………...36 1.6.4. OEA effects in the central nervous system …………………………………………37 1.7. Aim of the thesis …………………………………………………………………………..40