Application of Appel Reaction to the Primary Alcohol Groups of Fructooligosaccharides: Synthesis of 6,6′,6′′-Trihalogenated 1-Kestose Derivatives
Total Page:16
File Type:pdf, Size:1020Kb
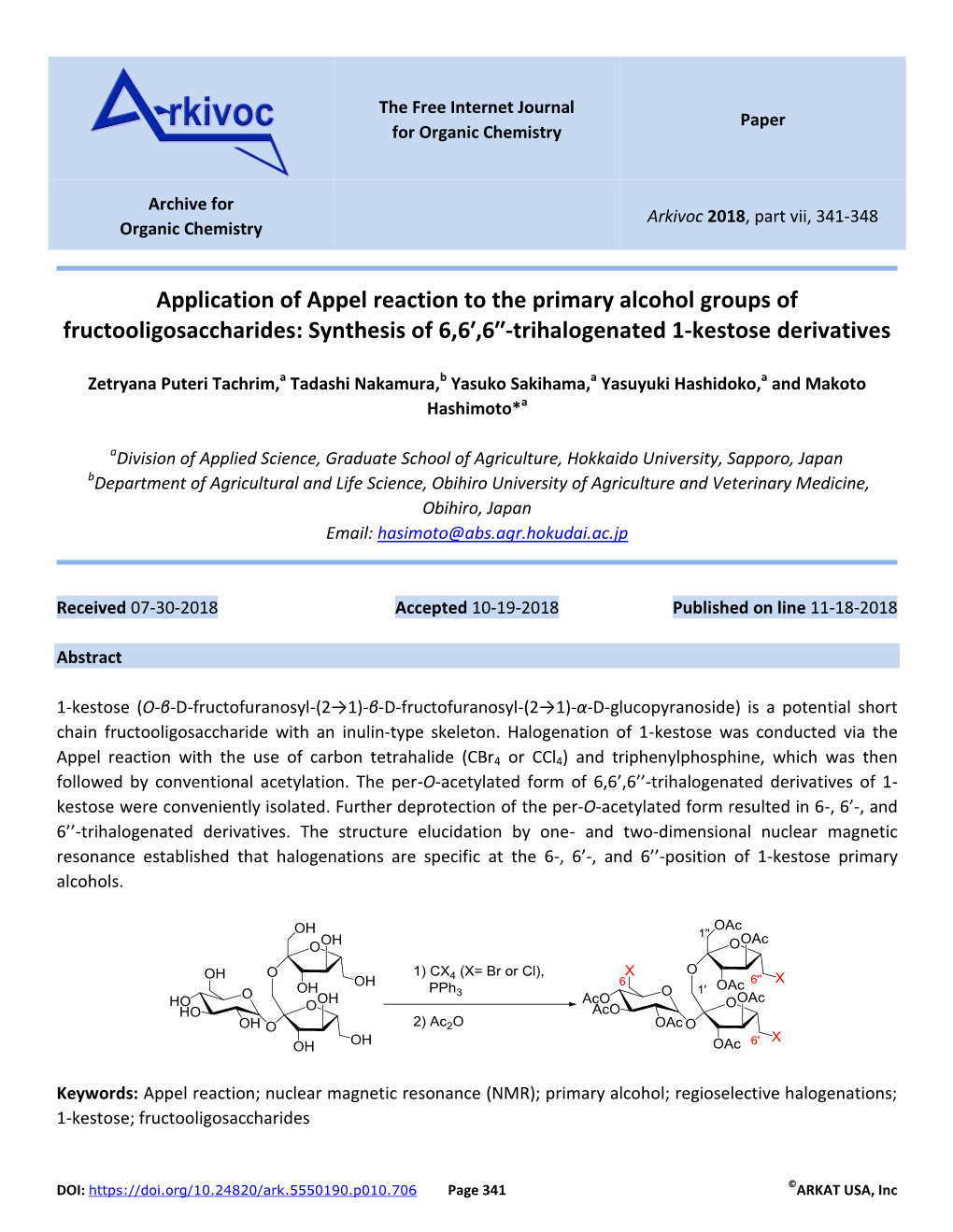
Load more
Recommended publications
-
Recent Syntheses of Steroidal Oxazoles, Oxazolines and Oxazolidines
A Platinum Open Access Journal Review for Organic Chemistry Free to Authors and Readers DOAJ Seal Arkivoc 2021, part i, 471-490 Recent syntheses of steroidal oxazoles, oxazolines and oxazolidines Besma Bendif,a,b Malika Ibrahim-Ouali,*a and Frédéric Dumur c aAix Marseille Univ, CNRS, Centrale Marseille, iSm2, F-13397 Marseille, France bLaboratoire de Chimie Appliquée, Faculté des Sciences, Université du 08 mai 1945 Guelma, Algeria cAix Marseille Univ, CNRS, ICR, UMR 72 73, F-13397 Marseille, France Email: [email protected] Received 03-15-2021 Accepted 04-11-2021 Published on line 05-08-2021 Abstract It was found that the introduction of heterocycles to steroids often leads in a change of their physiological activity and the appearance of new interesting biological precursors. Recent developments in the syntheses of steroidal oxazoles, oxazolines, and oxazolidines are described herein. The biological activities of those steroidal derivatives for which data are available are given. Keywords: Steroids, oxazoles, oxazolines, oxazolidines DOI: https://doi.org/10.24820/ark.5550190.p011.512 Page 471 ©AUTHOR(S) Arkivoc 2021, i, 471-490 Bendif, B. et al. Table of Contents 1. Introduction 2. Synthesis of Steroidal Oxazoles 3. Synthesis of Steroidal Oxazolines 4. Synthesis of Steroidal Oxazolidines 5. Conclusions Acknowledgements References 1. Introduction Steroids constitute an extensive and important class of biologically active polycyclic compounds that are widely used for therapeutic purposes.1-3 Even after decades of research, the total synthesis of steroid nuclei by improved strategies continues to receive considerable attention. Numerous methods have been exploited for the total synthesis of steroids which are widely distributed in nature and which possess practical medical importance. -
DEVELOPMENT of NOVEL METHODOLOGIES UTILIZING QUATERNARY AMMONIUM SALTS AS CATALYSTS by LINDSEY RAE CULLEN DISSERTATION Submitted
DEVELOPMENT OF NOVEL METHODOLOGIES UTILIZING QUATERNARY AMMONIUM SALTS AS CATALYSTS BY LINDSEY RAE CULLEN DISSERTATION Submitted in partial fulfillment of the requirements for the degree of Doctor of Philosophy in Chemistry in the Graduate College of the University of Illinois at Urbana-Champaign, 2015 Urbana, Illinois Doctoral Committee: Professor Scott E. Denmark, Chair Professor Martin D. Burke Professor Scott K. Silverman Professor Steven C. Zimmerman ii Abstract The first half of this thesis (Chapter 2) described the development of a fluoride-promoted conjugate addition of sulfur-stabilized carbanion nucleophiles to α,β-unsaturated ketones and esters. This reaction was achieved using a substoichiometric amount of TBAF, resulting in high yields on the desired 1,4-addition product. The addition of 1,3-dithianes was given particular focus as a novel method for the preparation of differentially protect 1,4-dicarbonyl compounds. Observation by 13C NMR spectroscopy provided evidence that the reaction proceeds through an ion pair, and attempts to extend this reaction to asymmetric additions using a chiral counterion are presented in detail. The second half of this thesis (Chapter 3) details development of a phase transfer catalyzed [2,3]-sigmatropic rearrangement of allyloxy carbonyl compounds. Initial investigation focused on identifying viable substrate classes that would undergo selective [2,3]-rearrangement under phase transfer conditions. Under certain conditions, the [2,3]-sigmatropic rearrangement of allyloxy carbonyl compounds takes place in the presence of a phase transfer agent, providing a rare example of a phase transfer catalyzed unimolecular reaction. In the course of this investigation it was found that catalysis is dependent on several variables including base concentration, catalyst structure, and substrate lipophilicity. -
Nickel-Catalyzed Cyanation of Benzylic and Allylic Pivalates
Nickel-Catalyzed Cyanation of Benzylic and Allylic Pivalates by Alexandria Daria Maria Jeanneret A thesis submitted in conformity with the requirements for the degree of Master of Science Department of Chemistry University of Toronto © Copyright by Alexandria Daria Maria Jeanneret 2018 Nickel-Catalyzed Cyanation of Benzylic and Allylic Pivalates Alexandria Daria Maria Jeanneret Master of Science Department of Chemistry University of Toronto 2018 Abstract Nitriles are considered very versatile functional groups due to their ability to easily be transformed into a variety of other functional groups in one or two steps. In particular, the synthesis of α-arylnitriles is of interest to organic chemists due to their presence in pharmaceuticals and their value as synthetic intermediates. Taking advantage of nickel’s unique ability to insert into a C‒O bond, the focus of this thesis is on the nickel-catalyzed cyanation of benzylic and allylic pivalates, exploring the use of inorganic and organic cyanide sources for this transformation. The substrate scope for the synthesis of benzylic and allylic nitriles will be presented as well as studies examining the functional group tolerance of this cyanation reaction, which led to further insights into the mechanism and applicability of this chemistry. ii Acknowledgments First and foremost, I would like to thank Professor Sophie Rousseaux for the opportunity to work to work in her lab over the last year. Her passion for chemistry is beyond contagious and her guidance has been invaluable. I would also like to thank Professor Mark Taylor for his help with this thesis. Secondly, I would like to thank all the staff at the NMR and AIMS facility for all their hard-work and dedication to ensuring the instruments run smoothly and for always taking the time to answer questions. -
Regioselective Synthesis of Enol Triflates by Enolate Trapping
Reviews and Accounts ARKIVOC 2012 (i) 432-490 The Hendrickson ‘POP’ reagent and analogues thereof: synthesis, structure, and application in organic synthesis Ziad Moussa Department of Chemistry, Taibah University, P.O. Box 30002, Almadinah Almunawarrah, Saudi Arabia E-mail: [email protected] DOI: http://dx.doi.org/10.3998/ark.5550190.0013.111 Abstract This comprehensive review discusses the synthesis, structure, and application of the Hendrickson ‘POP’ reagent and its analogues in organic synthesis. Specifically, the review describes applications of the preceding dehydrating agents in functional group interconversions, heterocycle synthesis, and total synthesis of natural products. Many examples are provided from the literature to show the scope and selectivity (regio and chemo) in these transformations. Keywords: Hendrickson ‘POP’ reagent, functional group interconversions, heterocycle synthesis, total synthesis of natural products Table of Contents 1. Introduction 2. The Hendrickson Reagent 2.1. Preparation of the Hendrickson reagent 2.2. Application of the Hendrickson reagent in functional group interconversions 2.2.1. Conversion of aldoximes into nitriles 2.2.2. Conversion of carboxylic acids to benzimidazole, benzoxazole, and benzthiazole heterocycles 2.2.3. Amide and amidine synthesis 2.2.4. Conversion of carboxylic acids into acid anhydrides 2.2.5. Ester formation from acid and alcohol 2.2.6. Conversion of activated ketones to alkynes 2.2.7. Cyclodehydrations of diols and amino alcohols 2.2.8. Conversion of epoxides to dienes 2.2.8.1 Mechanism of double elimination of epoxides to dienes Page 432 ©ARKAT-USA, Inc. Reviews and Accounts ARKIVOC 2012 (i) 432-490 2.2.9. Conversion of sulfonic acids to sulfonamides and activated sulfonate esters 2.2.10. -
A Biosynthetically Inspired Synthetic Route to Substituted Furans, and Its Application to the Total Synthesis of the Furan Fatty
A Biosynthetically Inspired Synthetic Route to Substituted Furans, and its Application to the Total Synthesis of the Furan Fatty Acid F5 A thesis submitted in partial fulfilment of the requirements of the degree Doctor of Philosophy Robert Jack Lee Supervisors: Dr Gareth J. Pritchard and Dr Marc C. Kimber 1 Thesis Abstract Dietary fish oil supplementation has long been shown to have significant health benefits, largely stemming from the anti-inflammatory activity of the ω-3 and ω-6 polyunsaturated fatty acids (PUFAs) present in fish oils. The anti-inflammatory properties of these fatty acids has been linked to beneficial health effects, such as protecting the heart, in individuals consuming diets rich in fish, or supplemented with fish oils.1 These effects are highly notable in the Māori people native to coastal regions of New Zealand; the significantly lower rates of heart problems compared to the inland populous has been attributed to the consumption of the green lipped mussel Perna Canaliculus. Commercially available health supplements based on the New Zealand green lipped mussel include a freeze-dried powder and a lipid extract (Lyprinol®), the latter of which has shown anti- inflammatory properties comparable to classical non-steroidal anti-inflammatory drugs (NSAIDs) such as Naproxen.2 GCMS analysis of Lyprinol by Murphy et al. showed the presence of a class of ω-4 and ω-6 PUFAs bearing a highly electron rich tri- or tetra-alkyl furan ring, which were designated furan fatty acids (F-acids).3 Due to their instability, isolation of F- acids from natural sources cannot be carried out and a general synthetic route toward this class of natural products was required. -
Activation of Alcohols Toward Nucleophilic Substitution: Conversion of Alcohols to Alkyl Halides Amani Atiyalla Abdugadar
University of Northern Colorado Scholarship & Creative Works @ Digital UNC Theses Student Research 12-1-2012 Activation of Alcohols Toward Nucleophilic Substitution: Conversion of Alcohols to Alkyl Halides Amani Atiyalla Abdugadar Follow this and additional works at: http://digscholarship.unco.edu/theses Recommended Citation Abdugadar, Amani Atiyalla, "Activation of Alcohols Toward Nucleophilic Substitution: Conversion of Alcohols to Alkyl Halides" (2012). Theses. Paper 22. This Text is brought to you for free and open access by the Student Research at Scholarship & Creative Works @ Digital UNC. It has been accepted for inclusion in Theses by an authorized administrator of Scholarship & Creative Works @ Digital UNC. For more information, please contact [email protected]. © 2012 Amani Abdugadar ALL RIGHTS RESERVED UNIVERSITY OF NORTHERN COLORADO Greeley, Colorado The Graduate School ACTIVATION OF ALCOHOLS TOWARD NEOCLEOPHILIC SUBSTITUTION: CONVERSION OF ALCOHOLS TO ALKYL HALIDES A Thesis Submitted in Partial Fulfillment of the Requirements for the Degree of Master of Science Amani Abdugadar College of Natural and Health Sciences Department of Chemistry and Biochemistry December, 2012 This Thesis by: Amani Abdugadar Entitled: Activation of Alcohols Toward Neocleophilic Substitution: Conversion of Alcohols to Alkyl Halides has been approved as meeting the requirement for the Master of Science in College of Natural and Health Sciences in Department of Chemistry and Biochemistry Accepted by the Thesis Committee ______________________________________________________ Michael D. Mosher, Ph.D., Research Co-Advisor ______________________________________________________ Richard W. Schwenz, Ph.D., Research Co-Advisor ______________________________________________________ David L. Pringle, Ph.D., Committee Member Accepted by the Graduate School _________________________________________________________ Linda L. Black, Ed.D., LPC Acting Dean of the Graduate School and International Admissions ABSTRACT Abdugadar, Amani. -
Recent Syntheses of Steroidal Oxazoles, Oxazolines and Oxazolidines Besma Bendif, Malika Ibrahim-Ouali, Frédéric Dumur
Recent syntheses of steroidal oxazoles, oxazolines and oxazolidines Besma Bendif, Malika Ibrahim-Ouali, Frédéric Dumur To cite this version: Besma Bendif, Malika Ibrahim-Ouali, Frédéric Dumur. Recent syntheses of steroidal oxazoles, oxa- zolines and oxazolidines. ARKIVOC - Online Journal of Organic Chemistry, Arkat USA Inc, 2021, 2021 (1), pp.471-490. 10.24820/ark.5550190.p011.512. hal-03230347 HAL Id: hal-03230347 https://hal.archives-ouvertes.fr/hal-03230347 Submitted on 19 May 2021 HAL is a multi-disciplinary open access L’archive ouverte pluridisciplinaire HAL, est archive for the deposit and dissemination of sci- destinée au dépôt et à la diffusion de documents entific research documents, whether they are pub- scientifiques de niveau recherche, publiés ou non, lished or not. The documents may come from émanant des établissements d’enseignement et de teaching and research institutions in France or recherche français ou étrangers, des laboratoires abroad, or from public or private research centers. publics ou privés. The Free Internet Journal Review for Organic Chemistry Archive for Arkivoc 2021, part i, 471-490 Organic Chemistry Recent syntheses of steroidal oxazoles, oxazolines and oxazolidines Besma Bendif,a,b Malika Ibrahim-Ouali,*a and Frédéric Dumur c aAix Marseille Univ, CNRS, Centrale Marseille, iSm2, F-13397 Marseille, France bLaboratoire de Chimie Organique Appliquée, Faculté des Sciences, Université de Guelma, Algeria cAix Marseille Univ, CNRS, ICR, UMR 7273, F-13397 Marseille, France Email address: [email protected] Received 03-15-2021 Accepted 04-11-2021 Published on line 05-08-2021 Abstract It was found that the introduction of heterocycles to steroids often leads in a change of their physiological activity and the appearance of new interesting biological precursors. -
Copyrighted Material
JWST960-SUBIND JWST960-Smith October 25, 2019 9:5 Printer Name: Trim: 254mm × 178mm SUBJECT INDEX The vast use of transition metal catalysts in organic chemistry makes the citation of every individual metal impractical, so there are limited citations of individual metals. Palladium is one exception where individual citations are common, in keeping with the widespread use of that metal. However, in most cases, the term metal catalyst, or catalyst, metal is used as a heading, usually representing transition metals. A-SE2 mechanism 893 and the steering wheel model acceleration of Diels-Alder reactions 487 151–152 reactions, high pressure A1 mechanism, acetal hydrolysis and universal NMR database 1038 487 155 hydrogen-bonding 1038 A1,3-strain 196 Cahn-Ingold-Prelog system hydrophobic effect 1038 A2 mechanism, acetal hydrolysis 149–152 in water 1038 487 determination 152 ionic liquids 1038 ab initio calculations 36 D/L nomenclature 149 micellular effects 1038 and acidity 346 Kishi’s NMR method 155 microwave irradiation 1038 and antiaromaticity 71 sequence rules 149–152 phosphate 1039 and nonclassical carbocations absolute hardness 64, 359, 361 solid state 1038 427 table 361 ultracentrifuge 1038 norbornyl carbocation 436 absorbents, chiral 168 ultrasound 1038 ab initio studies 248 absorption, and conjugation 317 zeolites 1038 1,2-alkyl shifts in alkyne anions differential, and diastereomers acceleration, Petasis reaction 1349 168 1202 and cubyl carbocation 413 differential, and resolution 169 acenaphthylene, reaction with and SN2 408–409 abstraction, -
The Application of a Monolithic Triphenylphosphine Reagent for Conducting Appel Reactions in Flow Microreactors
The application of a monolithic triphenylphosphine reagent for conducting Appel reactions in flow microreactors Kimberley A. Roper1, Heiko Lange1, Anastasios Polyzos1, Malcolm B. Berry2, Ian R. Baxendale1 and Steven V. Ley*1 Full Research Paper Open Access Address: Beilstein J. Org. Chem. 2011, 7, 1648–1655. 1Innovative Technology Centre, Department of Chemistry, University doi:10.3762/bjoc.7.194 of Cambridge, Lensfield Road, Cambridge, Cambridgeshire, CB2 1EW, UK and 2GlaxoSmithKline, Gunnels Wood Road, Stevenage, Received: 30 September 2011 Hertfordshire, SG1 2NY, UK Accepted: 16 November 2011 Published: 08 December 2011 Email: Steven V. Ley* - [email protected] This article is part of the Thematic Series "Chemistry in flow systems II". * Corresponding author Guest Editor: A. Kirschning Keywords: © 2011 Roper et al; licensee Beilstein-Institut. Appel reaction; bromination; flow chemistry; solid-supported reagent; License and terms: see end of document. triphenylphosphine monolith Abstract Herein we describe the application of a monolithic triphenylphosphine reagent to the Appel reaction in flow-chemistry processing, to generate various brominated products with high purity and in excellent yields, and with no requirement for further off-line puri- fication. Introduction Flow chemistry is well-established as a useful addition to the these can suffer from poor mass transfer as well as presenting toolbox of the modern research chemist, with advantages practical problems caused by the swelling or compression char- accrued through increased efficiency, reproducibility and reac- acteristics of the beads related to the solvent employed. To tion safety [1-6]. Further benefits can be realised when flow circumvent some of the issues with bead-type supports, mono- processing techniques are combined with the use of solid- liths have been developed as replacements for use in supported reagents and scavengers, which allow telescoping of continuous-flow synthesis. -
TCIMAIL No.157
ISSN 1349-4848 number157 CONTENTS 2 Contribution - Study of Ion-supported Reagents for Organic Synthesis: IS-Ph3P, IS-MSO, IS-MS, and IS-DIB Hideo Togo Professor of Graduate School of Science, Chiba University 10 Chemistry Chat –Focusing on the Elements- - Elements for the Fight against Cancer Kentaro Sato 14 Science “Spring” Seminar - Carbonyl Olenation (2) Takeshi Takeda Professor of Department of Applied Chemistry, Tokyo University of Agriculture and Technology 20 Short Topic: More ways to use reagents - Hydrolysis of Thioacetals Using Oxidizing Reagents Haruhiko Taguchi Tokyo Chemical Industry Co., Ltd. 24 New Products Information : - Ru-Re Supramolecular Complex Photocatalyst Capable of Eciently Reducing CO2 to CO - High Regioregular p-type Organic Semiconducting Polymer - Novel Aromatic Triuoromethylating Reagent - Homologation Reaction by Ketene Dithioacetalization - HDAC Inhibitor No.157 No.157 Study of Ion-supported Reagents for Organic Synthesis: IS-Ph3P, IS-MSO, IS-MS, and IS-DIB Hideo Togo Graduate School of Science, Chiba University 1. Introduction mixture and reuse of those ion-supported reagents for the same reactions, as shown in Fig. 1. The great advantages of these In organic synthesis, most reactions require organic ion-supported reagents are the simple isolation of the desired reagents and therefore, after each reaction, the desired product products by diethyl ether extraction of the reaction mixture must be purified by column chromatography to remove organic and subsequent removal of the solvent, and their recyclable co-products derived from organic reagents. In view of use for the same reactions through high recovery and efficient environmentally benign organic synthesis, novel ion-supported regeneration, and therefore those ion-supported reagents are reagents, such as ion-supported Ph3P (IS-Ph3P A and B), ion- user-friendly and environmentally benign. -
Aminooxy Reagents for Synthesis and Analysis : Expanding the Role of Oximation
University of Louisville ThinkIR: The University of Louisville's Institutional Repository Electronic Theses and Dissertations 5-2013 Aminooxy reagents for synthesis and analysis : expanding the role of oximation. Sebastien Laulhe University of Louisville Follow this and additional works at: https://ir.library.louisville.edu/etd Recommended Citation Laulhe, Sebastien, "Aminooxy reagents for synthesis and analysis : expanding the role of oximation." (2013). Electronic Theses and Dissertations. Paper 796. https://doi.org/10.18297/etd/796 This Doctoral Dissertation is brought to you for free and open access by ThinkIR: The University of Louisville's Institutional Repository. It has been accepted for inclusion in Electronic Theses and Dissertations by an authorized administrator of ThinkIR: The University of Louisville's Institutional Repository. This title appears here courtesy of the author, who has retained all other copyrights. For more information, please contact [email protected]. AMINOOXY REAGENTS FOR SYNTHESIS AND ANALYSIS: EXPANDING THE ROLE OF OXIMATION by Sébastien Laulhé B.S., Ecole Nationale Supérieure de Chimie de Montpellier, 2007 M.S., Ecole Nationale Supérieure de Chimie de Montpellier, 2009 M.S., University of Louisville, 2012 A Dissertation Submitted to the Faculty of the College of Arts & Sciences of the University of Louisville In Partial Fulfillment of the Requirements For the Degree of Doctor of Philosophy Department of Chemistry University of Louisville Louisville, Kentucky May 2013! ! ! ! ! ! ! ! ! ! ! ! ! ! ! ! ! ! ! ! ! ! ! ! ! ! ! ! ! ! ! ! ! ! ! ! ! ! ! ! ! ! ! ! ! ! ! ! AMINOOXY REAGENTS FOR SYNTHESIS AND ANALYSIS: EXPANDING THE ROLE OF OXIMATION by Sébastien Laulhé B.S., Ecole Nationale Supérieure de Chimie de Montpellier, 2007 M.S., Ecole Nationale Supérieure de Chimie de Montpellier, 2009 M.S., University of Louisville, 2012 A Dissertation Approved on April 17, 2013 by the Following Dissertation Committee: Thesis Director: Dr. -
Highly Stereoselective Bromination of Alkyl Phenyl Sulphides† Cite This: Chem
Chemical Science View Article Online EDGE ARTICLE View Journal | View Issue Sulphide as a leaving group: highly stereoselective bromination of alkyl phenyl sulphides† Cite this: Chem. Sci.,2019,10,9042 a a ab a All publication charges for this article Daniele Canestrari, Caterina Cioffi, Ilaria Biancofiore, Stefano Lancianesi, have been paid for by the Royal Society Lorenza Ghisu,a Manuel Ruether,c John O'Brien,c Mauro F. A. Adamo *a of Chemistry and Hasim Ibrahim *a A conceptionally novel nucleophilic substitution approach to synthetically important alkyl bromides is presented. Using molecular bromine (Br2), readily available secondary benzyl and tertiary alkyl phenyl sulphides are converted into the corresponding bromides under exceptionally mild, acid- and base-free reaction conditions. This simple transformation allows the isolation of elimination sensitive benzylic b- bromo carbonyl and nitrile compounds in mostly high yields and purities. Remarkably, protic functionalities such as acids and alcohols are tolerated. Enantioenriched benzylic b-sulphido esters, readily prepared by asymmetric sulpha-Michael addition, produce the corresponding inverted bromides with high stereoselectivities, approaching complete enantiospecificity at À40 C. Significantly, the Creative Commons Attribution 3.0 Unported Licence. Received 18th July 2019 reported benzylic b-bromo esters can be stored without racemisation for prolonged periods at À20 C. Accepted 7th August 2019 Their synthetic potential was demonstrated by the one-pot preparation of g-azido alcohol (S)-5 in 90% DOI: 10.1039/c9sc03560e ee. NMR studies revealed an initial formation of a sulphide bromine adduct, which in turn is in rsc.li/chemical-science equilibrium with a postulated dibromosulphurane intermediate that undergoes C–Br bond formation.