Monitoring and Multi-Messenger Astronomy with Icecube
Total Page:16
File Type:pdf, Size:1020Kb
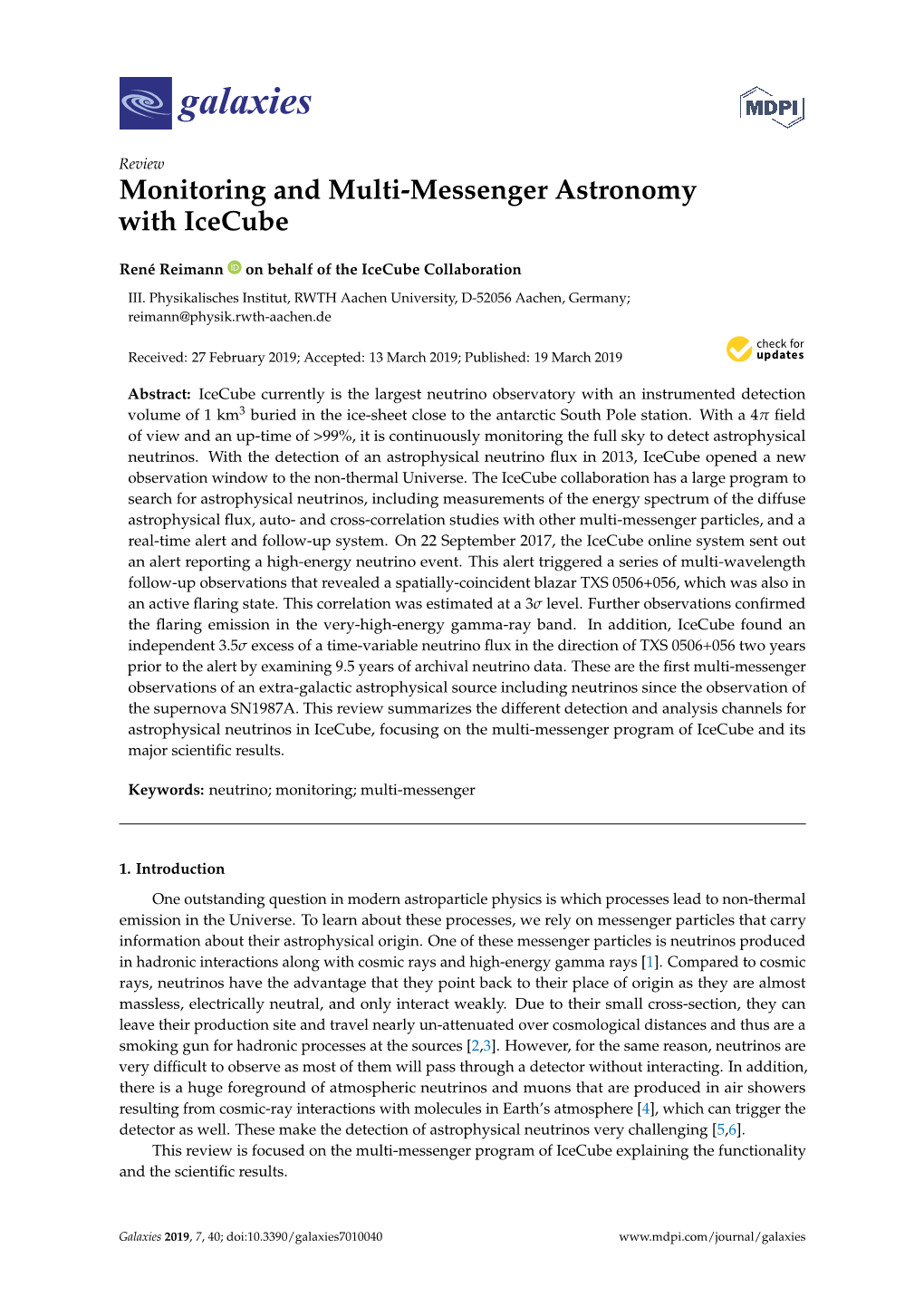
Load more
Recommended publications
-
Neutrino Astronomy
Astrophysics from Antarctica Proceedings IAU Symposium No. 288, 2012 c International Astronomical Union 2013 M. G. Burton, X. Cui & N. F. H. Tothill, eds. doi:10.1017/S1743921312016729 Neutrino Astronomy: An Update Francis Halzen Department of Physics and Wisconsin IceCube Particle Astrophysics Center, University of Wisconsin-Madison email: [email protected] Abstract. Detecting neutrinos associated with the still enigmatic sources of cosmic rays has reached a new watershed with the completion of IceCube, the first detector with sensitivity to the anticipated fluxes. In this review, we will briefly revisit the rationale for constructing kilometer-scale neutrino detectors and summarize the status of the field. Keywords. Neutrinos, cosmic rays, astrophysics 1. Introduction Soon after the 1956 observation of the neutrino (Reines, 1956), the idea emerged that it represented the ideal astronomical messenger. Neutrinos reach us from the edge of the Universe without absorption and with no deflection by magnetic fields. Neutrinos have the potential to escape unscathed from the inner neighborhood of black holes, and, the subject of this update, from the cosmic accelerators where cosmic rays are born. Their weak interactions also make cosmic neutrinos very difficult to detect. Immense parti- cle detectors are required to collect cosmic neutrinos in statistically significant numbers (Klein, 2008). Already by the 1970s, it had been understood that a kilometer-scale de- tector was needed to observe the “cosmogenic” neutrinos produced in the interactions of cosmic rays with background microwave photons (Roberts, 1992). Today’s estimates of the sensitivity for observing potential cosmic accelerators such as Galactic supernova remnants, active galactic nuclei (AGN), and gamma-ray bursts (GRB) unfortunately point to the same exigent requirement (Gaisser, 1995). -
Session: Neutrino Astronomy
Session: Neutrino Astronomy Chair: Takaaki Kajita, Institute for Cosmic Ray Research, Univ. of Tokyo Basic natures of neutrinos Neutrino was introduced in 1930 by W. Pauli in order to save the energy conservation law in nuclear beta decay processes, in which the emitted electron exhibits a continuous energy spectrum. It was assumed that the penetration power of neutrinos is much higher than that of the gamma rays. More than 20 years later, the existence of neutrinos was experimentally confirmed by an experiment that measured neutrinos produced by a nuclear power reactor. Since then, the basic nature of neutrinos has been understood through various theoretical and experimental studies: Neutrinos interact with matter extremely weakly. The number of neutrino species is three. They are called electron-neutrino, muon-neutrino and tau-neutrino. In addition, recent neutrino experiments discovered that neutrinos have very small masses. Observing the Universe by neutrinos (1) Because of the extremely high penetration power of neutrinos, neutrinos produced at the center of a star easily penetrate to the outer space. Theories of astrophysics predict that there are various processes that neutrinos play an essential role at the center of stars. For example, the Sun is generating its energy by nuclear fusion processes in the central region. In these processes, low energy electron neutrinos with various energy spectra are generated. Thus the observation of solar neutrinos directly probes the nuclear fusion reactions in the Sun. Another example is the supernova explosion. While the optical measurements observe an exploding star, what is happening in the central region of the star is the collapse of the core of a massive star. -
Multi-Messenger Astrophysics with the First Lines of the Km3net Neutrino Telescopes
MULTI-MESSENGER ASTROPHYSICS WITH THE FIRST LINES OF THE KM3NET NEUTRINO TELESCOPES PHD SUPERVISOR : THIERRY PRADIER (MAÎTRE DE CONFÉRENCES) INSTITUT PLURIDISCIPLINAIRE HUBERT CURIEN, DÉPARTEMENT RECHERCHES SUBATOMIQUES 23 RUE DU LOESS BP 28 67037 STRASBOURG CEDEX 2 TEL : 03 88 10 6620 ; E-MAIL : [email protected] The proposed PhD thesis will be performed in the framework of the european collaborations KM3NeT, which use underwater « neutrino telescopes » in the Mediterranean Sea, to detect and study GeV to PeV atmospheric/cosmic neutrinos. IceCube has revealed in 2013 the existence of cosmic neutrinos, yielding information on the origin of cosmic rays. In July 2018, a possible connection of these cosmic neutrinos with astrophysical sources such as blazars was revealed. Moreover, the observation of gravitational waves and electromagnetic radiations from the merger of two neutron stars in 2017, and the search for coincident neutrinos, has opened the field of multi-messenger astrophysics : the combination of photons from all wavelengths, neutrinos, cosmic-rays and gravitational waves to improve our knowledge of astrophysical sources and discover new sources, in which IPHC has taken an active part. In 2019, the next-generation neutrino telescopes built by the KM3NeT collaboration will begin their deployment and data taking, with more than 300 lines on 2 sites to be deployed over a few years. The french site in Toulon will be dedicated to low energy GeV neutrinos, to determine the unknown neutrino mass hierarchy, with ORCA (Oscillation Research with Cosmics in the Abyss). The italian site, in Sicily, will host ARCA (Astroparticle Research with Cosmics in the Abyss), dedicated, like ANTARES, to TeV-PeV neutrino astronomy. -
11 – Neutrino Astronomy
11 – Neutrino astronomy introduc)on to Astrophysics, C. Bertulani, Texas A&M-Commerce 1 11.1 – The standard solar model As we discussed in “stellar evolution III”, to obtain a reliable model for the sun, we need to solve four differential equations (in the absence of convection). dM(r) 2 (11.1) dP(r) GM(r)ρ(r) = 4πr ρ(r) = − (11.2) dr dr r2 dT(r) 3 (r) (r) dL(r) 2 (11.3) ρ κ (11.4) = 4πr ρ(r)ε(r) = − 2 3 L(r) dr dr 16πr σT (r) € € Complemented by P = P (, T, chemical composition)€ The equation of state € κ = κ (, T, chemical composition) Opacity ε = ε (, T, chemical composition) Energy generation But we also need to include how the chemical composition changes through nuclear reaction network. For the sun, the pp-chain and the CNO cycle are considered. introduc)on to Astrophysics, C. Bertulani, Texas A&M-Commerce 2 General reaction network When the reaction network proceeds via one-body and two-body reactions, such as the pp-chain. and CNO cycle. But 3-body processes such as the triple-alpha reaction are also possible. A general reaction network can be written as dYi i i = ∑N j λ jY j + ∑N jk ρNA < σ v > Y jYk + dt j jk (11.5) 2 i 2 ∑N jkm ρ NA < σ v > Y jYkYm +! jkm i where N j,k,l,... is the number of particles of nuclear species i created or destroyed by the reaction j + k + l + · · · à i. € The reactions listed on the right hand side of the equation above belong to three categories of reactions: (1) decays, photodisintegrations, electron and positron captures and neutrino induced reactions (rj = λjnj), (2) two-particle reactions (rj,k =< σv >j,knjnk), and (3) three-particle reactions 12 (rj,k,l =< σv>j,k,lnjnknl) like the triple-alpha process (α + α + α à C + γ). -
Gamma-Ray and Neutrino Astronomy
Sp.-V/AQuan/1999/10/07:19:58 Page 207 Chapter 10 γ -Ray and Neutrino Astronomy R.E. Lingenfelter and R.E. Rothschild 10.1 Continuum Emission Processes ............. 207 10.2 Line Emission Processes ................. 208 10.3 Scattering and Absorption Processes .......... 213 10.4 Astrophysical γ -Ray Observations ........... 216 10.5 Neutrinos in Astrophysics ................ 235 10.6 Current Neutrino Observatories ............. 237 10.1 CONTINUUM EMISSION PROCESSES Important processes for continuum emission at γ -ray energies are bremsstrahlung, magneto- bremsstrahlung, and Compton scattering of blackbody radiation by energetic electrons and positrons [1–6]. 10.1.1 Bremsstrahlung The bremsstrahlung luminosity spectrum of an optically thin thermal plasma of temperature T in a volume V is [3] 1/2 π 6 π 2 32 e 2 mc 2 L(ν)brem = Z neniVg(ν, T ) exp(−hν/kT), 3m2c4 3kT where the index of refraction is assumed to be unity, m is the electron mass, Z is the mean atomic 1/2 charge, ne and ni are the electron and ion densities, and the Gaunt factor g(ν, T ) ≈ (3kT/πhν) for hν>kT and T > 3.6 × 105 Z 2 K, or −38 2 −1/2 −1 −1 L(ν)brem ≈ 6.8 × 10 Z neniVg(ν, T )T exp(−hν/kT) erg s Hz . 207 Sp.-V/AQuan/1999/10/07:19:58 Page 208 208 / 10 γ -RAY AND NEUTRINO ASTRONOMY 10.1.2 Magnetobremsstrahlung The synchrotron luminosity spectrum of an isotropic, optically thin nonthermal distribution of −S relativistic electrons with a power-law spectrum, N(γ ) = N0γ , interacting with a homogeneous magnetic field of strength, H,is[5] . -
Dear Astronomers, What Is It That You Do in the Practice of Your Science?
Dear Astronomers, What is it that you do in the practice of your science? This email is an invitation to participate in a research study that explores the activities in which astronomers currently engage. The goal of the study is to characterize what it is that you do as a practicing astronomer. The results will provide educators, curriculum developers, and others a valuable tool as they work to create more authentic (real-world) experiences, improving astronomy learning for all. Participants in the survey must meet the following 4 requirements: • have one or more science or science related degrees, with at least one at the Masters Degree level or higher, • work primarily in one or more of the astronomy sub-disciplines (if you are unsure, please see definition of astronomy sub-disciplines at the end of this letter), • currently engaged in, or have engaged in, at least one astronomy related research project within the past two years, • and, be an astronomer primarily based in the United States, a U.S. territory, or a U.S. facility in another country. This research study is being conducted by Tim Spuck, a doctoral candidate in Curriculum and Instruction under the supervision of Dr. James Rye, professor in the West Virginia University College of Education and Human Services. This research study is part of a dissertation that is being conducted in partial fulfillment of the requirements of the Curriculum and Instruction Doctoral Program at West Virginia University. Your participation in this study is completely voluntary and consists of completing an online survey, which will take approximately 15 minutes. -
Index to JRASC Volumes 61-90 (PDF)
THE ROYAL ASTRONOMICAL SOCIETY OF CANADA GENERAL INDEX to the JOURNAL 1967–1996 Volumes 61 to 90 inclusive (including the NATIONAL NEWSLETTER, NATIONAL NEWSLETTER/BULLETIN, and BULLETIN) Compiled by Beverly Miskolczi and David Turner* * Editor of the Journal 1994–2000 Layout and Production by David Lane Published by and Copyright 2002 by The Royal Astronomical Society of Canada 136 Dupont Street Toronto, Ontario, M5R 1V2 Canada www.rasc.ca — [email protected] Table of Contents Preface ....................................................................................2 Volume Number Reference ...................................................3 Subject Index Reference ........................................................4 Subject Index ..........................................................................7 Author Index ..................................................................... 121 Abstracts of Papers Presented at Annual Meetings of the National Committee for Canada of the I.A.U. (1967–1970) and Canadian Astronomical Society (1971–1996) .......................................................................168 Abstracts of Papers Presented at the Annual General Assembly of the Royal Astronomical Society of Canada (1969–1996) ...........................................................207 JRASC Index (1967-1996) Page 1 PREFACE The last cumulative Index to the Journal, published in 1971, was compiled by Ruth J. Northcott and assembled for publication by Helen Sawyer Hogg. It included all articles published in the Journal during the interval 1932–1966, Volumes 26–60. In the intervening years the Journal has undergone a variety of changes. In 1970 the National Newsletter was published along with the Journal, being bound with the regular pages of the Journal. In 1978 the National Newsletter was physically separated but still included with the Journal, and in 1989 it became simply the Newsletter/Bulletin and in 1991 the Bulletin. That continued until the eventual merger of the two publications into the new Journal in 1997. -
Review/Preview of Neutrino Astronomy John G
XVIII International Workshop on Neutrino Telescopes 1990 18-22 March 2019, Venice, Italy Review/Preview of Neutrino Astronomy John G. Learned University of Hawaii I have quoted many slides from Amy Connelly at ARENA2018… excellent summary talk. Christian Spiering helped greatly with the tables of past, current and future projects. Our Dear Milla The Organizers asked me to make an update from the talk I gave about the “Future of Neutrino Astronomy” at Neutrino Telescopes 1990, amazingly to me 29 years ago, and it is a pleasure to be in Venice at this time when much has been accomplished in the neutrino business in such a time. Dear Milla asked for the 1990 talk, and it is to her I dedicate this (p)review. We all miss her gentle hand on guiding these ever stimulating meetings. But Mauro and friends are doing a great job. Milla Baldo Ceolin (1924-2011) plus ça change, plus c’est la même chose Map showing one interpretation of Yahweh (God) shows Moses the borders the Promised Land of the Promised (Frans Pourbus the Elder, c. 1565–80) (Wikipedia) Land, based on God's promise to Abraham (Genesis 15). (Wikipedia) But what they got was this…. Beginnings of HE Nu Astronomy • The journey to high energy neutrino astronomy starts with fantastic dreams in Russia and US in 1950’s with pioneers such as Moisei Markov, George Zatsepin, in Russia and Ken Greisen, and Fred Reines in the USA, and who first articulated the dream of detecting cosmic neutrinos, hopefully permitting us to view the universe in a very different light than photons. -
Astro-Ph/9810368V1 22 Oct 1998 Ye Frdainytdtce”Gesnsae Nhs16 Review
MADPH-98-1088 University of Wisconsin - Madison October 1998 LECTURES ON NEUTRINO ASTRONOMY: THEORY AND EXPERIMENT∗ FRANCIS HALZEN Department of Physics, University of Wisconsin, Madison, WI 53706 1. Overview of neutrino astronomy: multidisciplinary science. 2. Cosmic accelerators: the highest energy cosmic rays. 3. Neutrino beam dumps: supermassive black holes and gamma ray bursts. 4. Neutrino telescopes: water and ice. 5. Indirect dark matter detection. 6. Towards kilometer-scale detectors. 1 Neutrino Astronomy: Multidisciplinary Science Using optical sensors buried in the deep clear ice or deployed in deep ocean and lake waters, neutrino astronomers are attacking major problems in astronomy, astrophysics, cosmic ray physics and particle physics by commissioning first generation neutrino telescopes. Planning is already underway to instrument a cubic volume of ice or water, 1 kilometer on the side, as a detector of neutrinos in order to reach the effective telescope area of 1 kilometer squared which is, according to estimates covering a wide range of scientific objectives, required to address the most fundamental questions. This infrastructure provides unique opportunities for yet more interdisciplinary science covering the geosciences and biology. Among the many problems which high energy neutrino telescopes will address are the origin of cosmic rays, the engines which power active galaxies, the nature of gamma ray bursts, the search for the annihilation products of halo cold dark matter (WIMPS, supersymmetric particles(?)), galactic supernovae and, possibly, even the structure of Earth’s interior. Coincident experiments with Earth- and space-based gamma ray observatories, cosmic ray telescopes and gravitational wave detectors such as LIGO can be contemplated. With arXiv:astro-ph/9810368v1 22 Oct 1998 high-energy neutrino astrophysics we are poised to open a new window into space and back in time to the highest-energy processes in the Universe. -
Cosmic Rays, Neutrinos & Multi-Messenger Astronomy With
Physics News Cosmic rays, neutrinos & multi-messenger astronomy with IceCube M. Rameez1 and S. Sarkar2 1 Department of High Energy Physics, Tata Institute of Fundamental Research, Mumbai 400005, India 2 Department of Physics, University of Oxford, Oxford OX1 3PU, UK E-mail: [email protected], [email protected] Mohamed Rameez did his PhD (2016) at the University of Geneva on the IceCube experiment. Subsequently he was a Carlsberg Postdoctoral fellow at the Niels Bohr Institute, Copenhagen and is now a Reader in the Dept. of High Energy Physics at TIFR. His interests include the phenomenology of astroparticle physics and cosmology, as well as the statistical interpretation of empirical data. Subir Sarkar obtained his PhD (1982) at TIFR and was a Research Associate in the Cosmic Rays Group. Subsequently he held visiting positions at CERN, Rutherford Laboratory and SISSA and since 1990 he has been a staff member at Oxford, where he was head of the Particle Theory Group (2011–19). He is also involved in astroparticle experiments, including the Pierre Auger Observatory (2003-13), IceCube Neutrino Observatory (2004–) and Cherenkov Telescope Array (2010–). Abstract The past decade has witnessed the opening up of a new window on to the Universe with the coming of age of high energy neutrino astronomy. The IceCube experiment at the South Pole discovered a diffuse flux of astrophysical neutrinos at TeV-PeV energies, and also identified the first candidate source for this flux: a flaring blazar 5.7 billion ly away. This was a breakthrough in real-time multi-messenger astronomy and has thrown up significant challenges to astrophysical models of such active galactic nuclei. -
Astronomy and Astrophysics with Neutrinos
IceCube: An Instrument for Neutrino Astronomy Francis Halzen1 and Spencer R. Klein2, 3 1Department of Physics, University of Wisconsin, 1150 University Avenue, Madison WI 53706 2Nuclear Science Division, Lawrence Berkeley National Laboratory, Berkeley, CA, 94720 3Department of Physics, University of California, Berkeley, CA, 94720 Abstract Neutrino astronomy beyond the Sun was first imagined in the late 1950s; by the 1970s, it was realized that kilometer-scale neutrino detectors were required. The first such instrument, IceCube, is near completion and taking data. The IceCube project transforms a cubic kilometer of deep and ultra-transparent Antarctic ice into a particle detector. A total of 5,160 optical sensors are embedded into a gigaton of Antarctic ice to detect the Cherenkov light emitted by secondary particles produced when neutrinos interact with nuclei in the ice. Each optical sensor is a complete data acquisition system, including a phototube, digitization electronics, control and trigger systems and LEDs for calibration. The light patterns reveal the type (flavor) of neutrino interaction and the energy and direction of the neutrino, making neutrino astronomy possible. The scientific missions of IceCube include such varied tasks as the search for sources of cosmic rays, the observation of Galactic supernova explosions, the search for dark matter, and the study of the neutrinos themselves. These reach energies well beyond those produced with accelerator beams. The outline of this review is as follows: • Neutrino Astronomy and Kilometer-Scale Detectors • High-Energy Neutrino Telescopes: Methodologies of Neutrino Detection • IceCube Hardware • High-Energy Neutrino Telescopes: Beyond Astronomy • Future Projects I. Introduction The Technology Soon after the 1956 observation of the neutrino1, the idea emerged that it represented the ideal astronomical messenger. -
Annual Report 1965
THE ROYAL ASTRONOMICAL SOCIETY OF CANADA ANNUAL REPORT 1965 SUPPLEMENT TO THE JOURNAL March 1966 STATEMENT OF POLICY OF THE EDITING COMMITTEE The Society renders a valuable service to astronomy by publishing a JOURNAL which has international recognition through its circulation to astronomers in many countries. Our JOURNAL provides Canadian scientists at the various observatories, government departments and universities with a suitable means of publishing certain of their research results. Indeed it is for this reason that the Government of Canada makes an annual grant of a substantial amount to the Society; in addition, various departments in the federal service, as well as the universities, effectively subsidize this publication by paying page charges and purchasing reprints. It is important, therefore, that the JOURNAL continues its tradition of publishing discoveries and original researches in astronomy from these and other sources. The JOURNAL must also be of value to the non-professional astronomer who is not concerned with the technical details of research but wishes to be informed on progress and activity in astronomy. These two primary aims to provide a means of publication for the professional astronomer and to keep the non-professional astronomer well informed, can be achieved only through a careful selection by the Editor of the material which appears in each number. To accomplish these two objectives, a policy was adopted when the JOURNAL was founded and has been closely followed by the successive Editors. A statement of policy was published in the 1962 Supplement to the JOURNAL; the Editing Com mittee believes that a revised statement should now be presented.