The Molecular Structure of Future Fuels
Total Page:16
File Type:pdf, Size:1020Kb
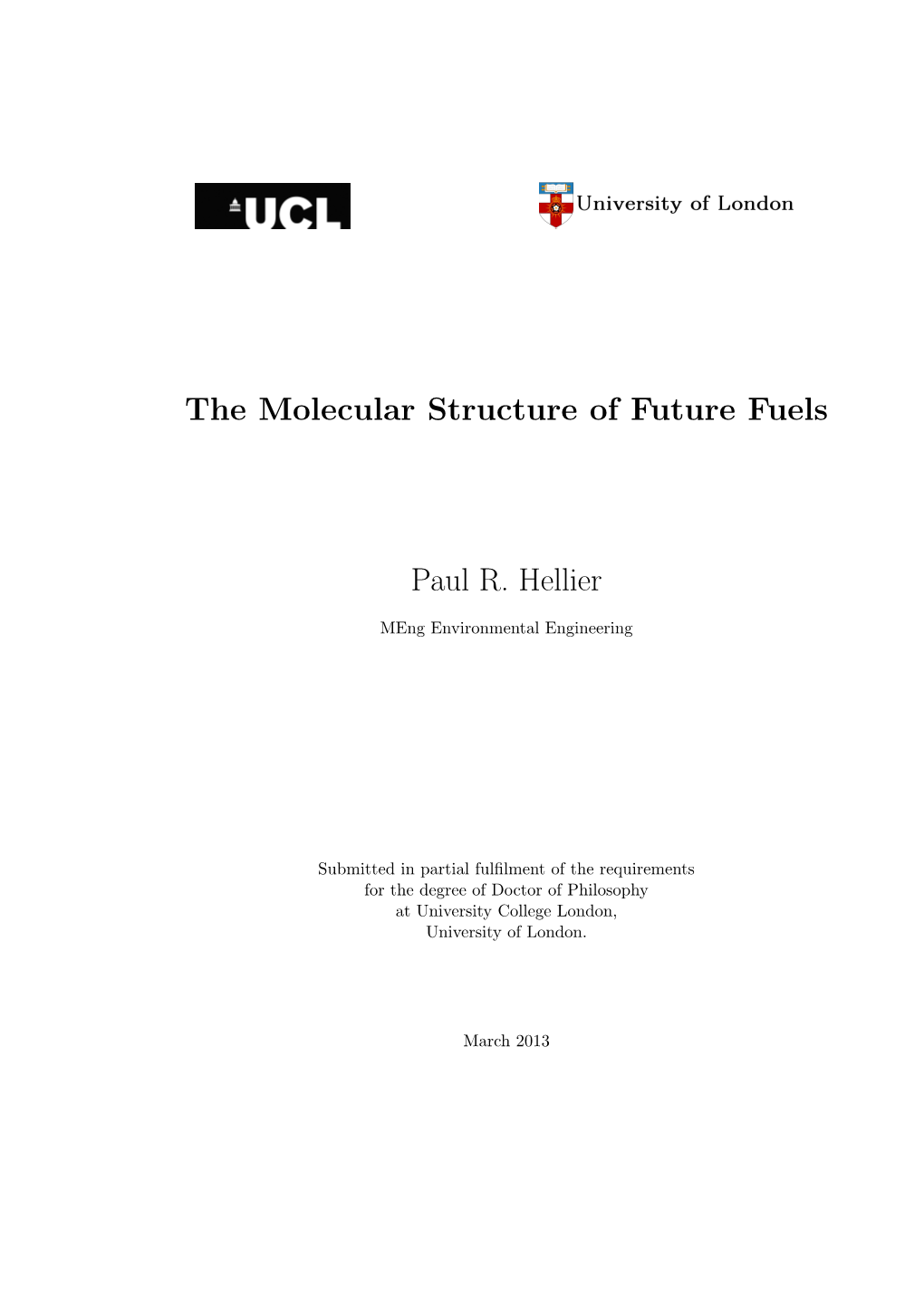
Load more
Recommended publications
-
Keynote and Plenary Speakers Speaker Biographies
AIChE Midwest Regional Conference March 13-14, 2018 – Chicago, IL Keynote and Plenary Speakers Tuesday, March 13, 2018 Jim Rekoske, Chief Technology Officer, UOP/Honeywell 8:15-9:30am – Sponsored by: Refining & Petrochemical Processing Russell A. Ogle, Principal Engineer, Exponent 12:45-1:45pm – Sponsored by: Process Safety and Occupational Health Christopher W. Jones, Professor and Associate Vice President for Research, Georgia Tech 12:45-1:45pm – Sponsored by: Catalysis & Reaction Engineering Wednesday, March 14, 2018 John A. Rodgers, Professor, Northwestern University 8:15-9:30am – Sponsored by: Biomedical, Pharmaceutical & Nano-Engineering Thomas Foust, Director, National Bioenergy Center, NREL 8:15-9:30am – Sponsored by: Energy and Sustainability Kimberly Grey, Professor and Chair, Civil and Environmental Engineering, Northwestern University 12:45-1:45pm – Sponsored by: Environmental Compliance & Remediation Doraiswami Ramkrishna, Distinguished Professor, Purdue University 6:30-7:30pm – Sponsored by: Process Engineering, Modeling, Optimization & Control and Fluid Properties, Fluid Dynamics & Transport Phenomena and Process Engineering, Modeling, Optimization & Control Speaker Biographies Tuesday Morning Keynote: 8:15 AM March 13, 2018 Jim Rekoske, Chief Technology Officer, UOP/Honeywell Presentation Title (tentative): Light Hydrocarbon Conversion Chemistry Challenges in an Era of Abundant Supply of Raw Material Biographical Sketch: Jim Rekoske is Vice President and Chief Technology Officer at UOP Honeywell. In this role, he is responsible for the entire technology organization, ranging from basic and applied research on new materials, catalysts, membranes and adsorbents through to process development, scale-up and commercialization. Previously, Jim served as Technical Director for Petrochemical Catalysts, Director of Technology for Universal Pharma Technologies and Vice President & General Manager of UOP’s Renewable Energy & Chemicals business unit. -
NACS Newsletter
Matt Neurock is the recipient of the 2015 Robert Burwell Club News Lectureship in Catalysis 24th NAM June 14-19, 2015 Club Directory NACS Pittsburgh, Pennsylvania Newsletter VOLUME XLIX, ISSUE 2 WWW.NACATSOC.ORG Back to Cover NACS Newsletter National Officers: President - one or more areas in the field of cataly- routes that prevail at the high surface Enrique Iglesia, University of Matt Neurock is the recipi- California-Berkeley; Vice- sis with emphasis on discovery and un- coverages relevant to catalytic practice, President - Bruce R. Cook,BP ent of the 2015 Robert Bur- Products NA, Inc.; Secretary - derstanding of catalytic phenomena, Hong-Xin Li, Zeolyst Internation- the direct participation of protic media al; Treasurer - C. Y. Chen, Chev- well Lectureship in Catalysis catalytic reaction mechanisms and as a co-catalyst, and the role of acid- ron Energy Technology Co.; Lead Trustee - Thomas F. identification and description of cata- base sites formed by hydroxyl interme- Degnan, University of Notre Dame; Communications Director lytic sites and species. diates on metals. His effective collabo- - Edrick Morales, Sasol Chemi- am pleased to an- cals (USA) LLC; Archivist - Uschi nounce that Professor Professor Matthew Neurock is be- rations with experimental groups have Graham, Topasol LLC. I ing recognized for his seminal contribu- led to fundamental and practical in- Club Representatives: Canada - Matthew Neurock of the Josephine Hill, University of tions to the development and applica- sights into the mechanisms of alkane Calgary; Chicago - Christopher L. University of Minnesota is Marshall, Argonne National La- the recipient of the2015 tion of theoretical and computational activation, Fischer-Tropsch synthesis, boratory; Mexico - José Antonio de los Reyes, Universidad Au- Robert Burwell Lecture- methods to elucidate catalytic mecha- selective oxidation and hydrogenation tonoma Metropolitana, Campus Iztapalapa; Michigan - Eric ship in Catalysis of the nisms and the active sites involved. -
CHEMICAL HERITAGE FOUNDATION GEORGE A. OLAH Transcript
CHEMICAL HERITAGE FOUNDATION GEORGE A. OLAH Transcript of an Interview Conducted by James G. Traynham and Arnold Thackray at Loker Hydrocarbon Research Institute Los Angeles, California on 3 February 2000 (With Subsequent Corrections and Additions) This interview has been designated as Free Access. One may view, quote from, cite, or reproduce the oral history with the permission of CHF. Please note: Users citing this interview for purposes of publication are obliged under the terms of the Chemical Heritage Foundation Oral History Program to credit CHF using the format below: George A. Olah, interview by James G. Traynham and Arnold Thackray at Loker Hydrocarbon Research Institute, Los Angeles, California, 3 February 2000 (Philadelphia: Chemical Heritage Foundation, Oral History Transcript # 0190). Chemical Heritage Foundation Oral History Program 315 Chestnut Street Philadelphia, Pennsylvania 19106 GEORGE A. OLAH 1927 Born in Budapest, Hungary, on 22 May Education 1945 B.S., organic chemistry, Technical University, Budapest 1949 Ph.D., organic chemistry, Technical University, Budapest Professional Experience Technical University, Budapest, Hungary 1949-1954 Assistant Professor to Associate Professor of Organic Chemistry Hungarian Academy of Sciences 1954-1956 Head of Department of Organic Chemistry and Associate Scientific Director of Central Research Institute The Dow Chemical Company 1957-1964 Senior Research Scientist Case Western Reserve University 1965-1967 Professor and Chairman, Department of Chemistry 1967-1969 Chairman of Combined Departments of Chemistry (Case Institute and Western Reserve University) 1967-1977 C. F. Mabery Distinguished Professor of Research in Chemistry University of Southern California 1977-1977 Professor of Chemistry and Scientific Director, Hydrocarbon Research Institute 1980-present Distinguished Professor of Chemistry 1983-present Donald P. -
2002 Issue 3
The North American Catalysis Society NEWSLETTER December, 2002 http://www.nacatsoc.org Vol. XXXVI No. 3 Emmett Award to Francisco Zaera The Paul H. Emmett Award in Fundamental Catalysis to Professor Francisco Zaera of the University of California at Riverside, USA. The award is sponsored by the Davison Chemical Division of W.R. Grace and Company. The Award is intended to recognize and encourage individual contributions (under the age of 45) in the field of catalysis with emphasis on discovery and understanding of catalytic phenomena, proposal of catalytic reaction mechanisms and identification of and description of catalytic sites and species. Professor Zaera’s main interests lie with the study of mechanisms of surface reactions by using modern surface- sensitive techniques. He is noted for bridging the knowledge on surface reactions with that of organometallic systems and for his extension of kinetic theories to reactions on surfaces. His nominators commented that he has placed particular emphasis on making a connection between the atomic details of surface reactions and heterogeneous catalytic processes. While most surface kinetic concepts have been recognized for some time, Francisco is credited with quantifying the kinetic consequences of these effects by a variety of surface science techniques to rationalize the rates observed in model systems and correlate them with practical heterogeneous catalysis rates. He has been given credit for unequivocally establishing that most hydrocarbon processing catalysts are covered with a carbonaceous layer during the catalytic process. By performing isotope labeling experiments and using vibrational spectroscopy and molecular beam studies, Professor Zaera determined that those deposits are not direct intermediates in hydrogenation-dehydrogenation steps, but rather an play an indirect role by tempering the high activity of the metal surfaces and providing a reservoir for the surface hydrogen. -
EI Magazine Summer 09.Indd
ENERGY INNOVATION OneOOne off Penn State Institutes of Energy and the Environment Energy Innovation Summer 2009 Energy Innovation is an annual publication from the EMS Energy Institute in the College of Earth and Mineral Sciences. Th e EMS Energy Institute is a leading research and development organization focused on energy science and engineering EMS Energy Institute Th e Pennsylvania State University C-211 Coal Utilization Laboratory Table of Contents University Park, PA 16802 Phone: 814.863.1333 Letter from the Director .....................................................3 Fax: 814.863.7432 www.energy.psu.edu Remarks from the Dean ....................................................4 Director Chunshan Song Features Associate Director Bruce Miller Research Partners within Penn State ..................................5 Writer/Editor Current Research Programs ...............................................6 Shea Winton Graphic Designer Students in Research .........................................................12 Elizabeth Wood Penn State and Chevron ....................................................14 [email protected] Questions regarding this publication National Energy Prize .......................................................16 [email protected] PSIEE Incorporates Energy .................................................17 Questions about the EMS Energy Institute Public and Private Partnerships .........................................18 Th is publication is available in alternative media Outreach ..........................................................................20 -
VLADIMIR NIKOLAEVICH IPATIEFF * November 21, 1867-November 29, 1952
NATIONAL ACADEMY OF SCIENCES V LADIMIR NIKOLAEVICH I PATIEFF 1867—1952 A Biographical Memoir by LE W I S S CHMERLING Any opinions expressed in this memoir are those of the author(s) and do not necessarily reflect the views of the National Academy of Sciences. Biographical Memoir COPYRIGHT 1975 NATIONAL ACADEMY OF SCIENCES WASHINGTON D.C. VLADIMIR NIKOLAEVICH IPATIEFF * November 21, 1867-November 29, 1952 BY LOUIS SCHMERLING ORTUNATELY FOR BOTH the scientific and the industrial F worlds, Vladimir Nikolaevich Ipatieff, who was born in Moscow on November 21, 1867, did not maintain his original intent to have a military career. When he was eleven years old, he was enrolled at the Third Moscow Military Gymnasium after three years in a classical gymnasium. He had no difficulty completing the courses, but his grades were poor until he was promoted to the sixth class at the age of fourteen. His favorite subject was mathematics, which he studied beyond the class requirements. His report card showed steady improvement, particularly in science courses. However, on being graduated at the age of sixteen, his application to the Mikhail Artillery School in St. Petersburg was rejected on the basis of his grades. He entered the Alexander Military School in Moscow, where he received an intense military education. He ranked near the top of his class and, rather than accept rank as sergeant, he decided to transfer in September 1886 to the Mikhail Artillery School, to which he was now admitted, the 450 ruble tuition being waived because he had the highest grades in his class in mechanics, artillery, and chemistry. -
United States Patent Office Patented Oct
2,721,885 United States Patent Office Patented Oct. 25, 1955 1. 2 thus a part of a saturated group including an alkyl group 2,721,885 and a cycloalkyl group, and an aralkyl group containing CONDENSATION OF AROMATIC COMPOUNDS no olefinic unsaturation. WITH UNSATURATED ORGANIC COMPOUNDS An object of this invention is to react an unsaturated or N THE PRESENCE OF COMPOSITE CATALYSTS 5 ganic compound with an aromatic compound selected from Herman Pines, Evanston, Ill., and Vladimir N. patieff, the group consisting of carbocyclic and heterocyclic aro deceased, late of Chicago, Ill., by Vladimir Haensel, matic ring compounds having attached to a nuclear carbon Hinsdale, Herman Pines, Evanston, and Vincetta atom a nonolefinic or saturated carbon atom to which is Kibort, Chicago, Ill., executors, assignors to Universal attached at least one hydrogen atom. Oil Products Company, Des Piaines, Ill., a corporation An additional object of this invention is to react a of Delaware monoolefin with an alkylaromatic hydrocarbon to form an aromatic hydrocarbon with a longer alkyl group. No Drawing. Application June 7, 1954, Another object of this invention is to condense ethylene Serial No. 435,031 with the side chain of an alkylaromatic hydrocarbon hav 14 Claims. (C. 260-668) ing attached to a nuclear carbon atom a carbon atom of said alkyl group to which is attached at least one hydro This application is a continuation-in-part of our co gen aton. pending application Serial No. 219,314, filed April 4, 1951, Still another object of this invention is to condense ethyl now abandoned. ene with the alkyl side chain of an alkylbenzene hydro This invention relates to the condensation of unsatu carbon, said side chain containing an alpha carbon atom rated organic compounds with aromatic compounds and to to which is attached a replaceabie hydrogen atom. -
Jingguang G. Chen (Curriculum Vitae)
Jingguang G. Chen (Curriculum Vitae) Current Positions: Chair and Thayer Lindsley Professor of Chemical Engineering, Columbia University, New York, NY 10027 Senior Chemist, Chemistry Division, Brookhaven National Laboratory Positions Held: 2019 – Present: Department Chair of Chemical Engineering, Columbia University 2012 – Present: Thayer Lindsley Professor of Chemical Engineering, Columbia University 2012 – Present: Joint Appointment at Chemistry Division, Brookhaven National Laboratory 2008 – 2012: Claire D. LeClaire Professor of Chemical Engineering, University of Delaware 2009 – 2012: Co-Director, Energy Frontier Research Center on Biomass Conversion 2008 – 2010: Interim Director, University of Delaware Energy Institute 2000 – 2007: Director, Center for Catalytic Science and Technology (CCST) 2005 – 2012: Professor of Chemistry (courtesy appointment), University of Delaware 2002 – 2012: Professor of Chemical Engineering, University of Delaware 2002 – 2005: Professor of Materials Science and Engineering, University of Delaware 1998 – 2001: Associate Professor of Materials Science and Engineering and Chemical Engineering, University of Delaware 1994 – 1998: Spokesperson of Exxon U1A Beamline at National Synchrotron Light Source 1990 – 1998: Staff Scientist, Exxon Corporate Research Laboratory, Annandale, New Jersey Selected Awards and Recognitions: Robert H. Wilhelm Award in Chemical Reaction Engineering, AIChE (2020) R.B. Anderson Award, Canadian Catalysis Division (2020) Distinguished Alumni Award, Chemistry Department, University -
Jingguang G. Chen (Curriculum Vitae)
Jingguang G. Chen (Curriculum Vitae) Current Positions: Thayer Lindsley Professor of Chemical Engineering, Columbia University, New York, NY 10027 Senior Chemist, Chemistry Department, Brookhaven National Lab Positions Held: 2012 – Present: Thayer Lindsley Professor of Chemical Engineering, Columbia University 2012 – Present: Joint Appointment at Chemistry Department, Brookhaven National Lab 2013 – Present: Guest Professor, Chemical Engineering, Tsinghua University, China 2012 – Present: Adjunct Professor, Chemical Engineering, University of Delaware 2008 – 2012: Claire D. LeClaire Professor of Chemical Engineering, University of Delaware 2009 – 2012: Co-Director, Energy Frontier Research Center on Biomass Conversion 2005 – 2012: Professor of Chemistry (courtesy appointment), University of Delaware 2008 – 2010: Interim Director, University of Delaware Energy Institute 2000 – 2007: Director, Center for Catalytic Science and Technology (CCST) 2002 – 2007: Professor of Chemical Engineering, University of Delaware 2002 – 2005: Professor of Materials Science and Engineering (courtesy appointment) 1998 – 2001: Associate Professor of Materials Science and Engineering and Chemical Engineering, University of Delaware 1994 – 1998: Spokesperson for Exxon U1A Beamline at Brookhaven National Laboratory 1990 – 1998: Staff Scientist, Exxon Corporate Research Laboratory, Annandale, New Jersey Selected Service in Catalysis and Energy Communities: Chair: Catalysis Division of American Chemical Society (2014 – Present) Co-Founder and Team Leader: Synchrotron -
2004 Issue 2
The North American Catalysis Society NEWSLETTER December, 2004 www.nacatsoc.org Vol. XXXVIII No. 2 Professor Matthew Neurock Selected as 2005 Emmett Awardee The North American Catalysis Society announced that Professor Matthew Neurock has been selected for the 2005 Paul H. Emmett Awardee in Fundamental Catalysis. The award consists of a plaque and a prize. The purpose of the Award is to recognize and encourage individual contributions (under the age of 45) in the field of catalysis with emphasis on discovery and understanding of catalytic phenomena, proposal of catalytic reaction mechanisms and identification of and description of catalytic sites and species. Professor Neurock’s interests include computational heterogeneous catalysis, molecular modeling, and kinetics of complex reaction systems. “Matt is recognized for his pioneering contributions to theoretical methods for the analysis and prediction of catalytic rates and selectivities. Matt has developed and applied theory and atomic - scale simulation in concerted and well-constructed efforts aimed at the elucidation of catalytic reaction mechanisms on metal and oxide surfaces and at understanding and designing active sites as they exist in realistic and complex reaction environments. He and his group have brought ab initio quantum mechanical methods together with kinetic Monte Carlo methods to simulate catalytic performance and the effects of the explicit reaction environment. His studies have brought fundamental insights into the roles of surface structure, crystallite size, surface coverage, alloying, condensed media, and transient intermediates.” Others remark that “Matt has been extremely successful at applying quantum chemical methods to a broad range of problems in surface chemistry.” Matt will give a plenary lecture and be recognized at the Spring 2005 North American Catalysis Society meeting in Philadelphia. -
A History of Industrial Catalysis
Catalysis Today 163 (2011) 3–9 Contents lists available at ScienceDirect Catalysis Today journal homepage: www.elsevier.com/locate/cattod A history of industrial catalysis John N. Armor * GlobalCatalysis.com L.L.C., 1608 Barkwood Dr., Orefield, PA 18069, USA ARTICLE INFO ABSTRACT Article history: Histories of catalysis have been told by others from different perspectives. This manuscript highlights Available online 12 January 2010 key catalytic discoveries that led to commercialized, industrial processes. The intent to show how catalysis evolved over the last 250 years into major industries focused not only at catalyst production, Keywords: but also significantly impacting the production of commodity, specialty and fine chemicals, as well as Biomass petrochemical, petroleum, emissions control, and polymerization. For centuries before 1750, catalysts Sustainability were used to make beverages and foods. One sees that the Lead Chamber process for the production of Emissions control sulfuric acid is among the earliest of catalytic processes and reaches back to 1750. Pursuit of a sound Energy fundamental understanding of catalysis in the 19th century, led to the application of these materials to a Zeolites Industrial catalysis variety of basic chemicals. The development of petroleum fuels led to a vast petrochemicals business History which in turn fed a growth in specialty and performance chemicals. New drivers in the 20th century from Chemical and petrochemical processes the transportation and the environmental business sectors provided market pull to bring about more catalytic solutions for more industries. The often novel, catalytic properties of zeolites created new commercial applications, while environmental legislation created market pull to use catalysis to meet the new regulatory standards. -
Herman Bloch
NATIONAL ACADEMY OF SCIENCES HERMAN SAMUEL BLOCH 1912–1990 A Biographical Memoir by JAMES P. SHOFFNER Any opinions expressed in this memoir are those of the author and do not necessarily reflect the views of the National Academy of Sciences. Biographical Memoirs, VOLUME 87 PUBLISHED 2005 BY THE NATIONAL ACADEMIES PRESS WASHINGTON, D.C. HERMAN SAMUEL BLOCH June 15, 1912–June 16, 1990 BY JAMES P. SHOFFNER ERMAN BLOCH WAS BORN in Chicago to Aaron and Esther H Bloch, who were immigrants from the Ukraine. He attended Senn High School on Chicago’s north side, gradu- ating in 1929 with high honors. According to his principal, his grade point average of 97.41 was the highest ever achieved by a Senn graduate. On the basis of this outstanding record he was granted a full-tuition scholarship to the University of Chicago, where he graduated Phi Beta Kappa in 1933, with a degree in chemistry and physics. He continued at the University of Chicago for graduate school, receiving his doctorate in organic chemistry in 1936, with Prof. Julius Stieglitz as his thesis advisor. After receiving his doctorate he joined Universal Oil Products Co., a research company specializing in the devel- opment of refining processes and products for the petroleum industry. In 1940 he married Elaine Judith Kahn, also a University of Chicago graduate. Herman and Judith had three children, Aaron, Janet L., and Merry D., of whom I will say more later. Herman joined Universal Oil Products (UOP) during a period when the petroleum industry—while not exactly in its infancy—certainly was not that far advanced technologi- cally.