Deciphering Cytoskeletal Junctional Interactions During Blood Vessel Morphogenesis in Vivo
Total Page:16
File Type:pdf, Size:1020Kb
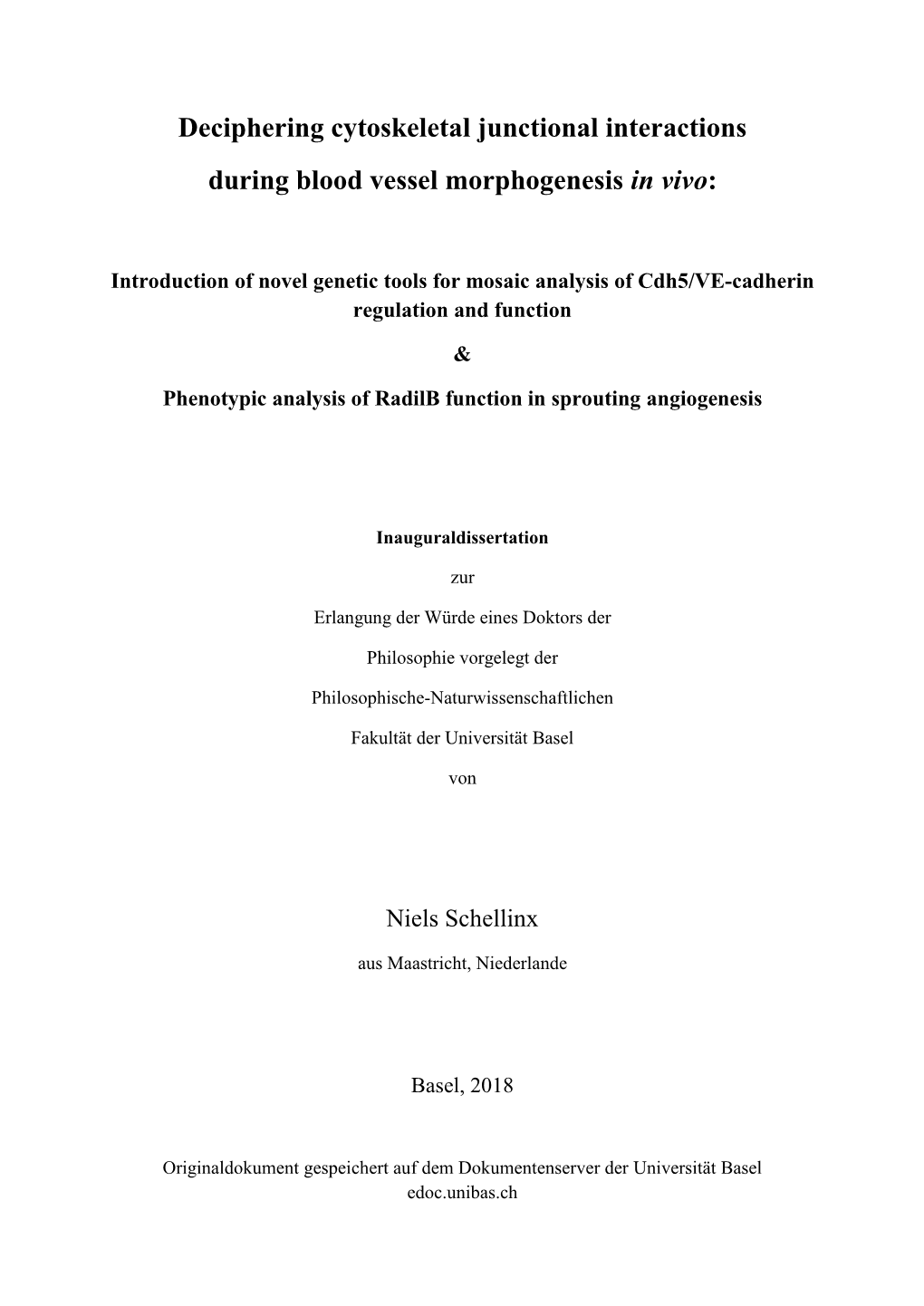
Load more
Recommended publications
-
Swine in Biomedical Research Conference 2005
Swine in Biomedical Research Conference 2005 www.swinegenomics.com Organizing Committee: Lawrence B. Schook, Ph.D. (Chair) Dept. Animal Sciences, Veterinary Pathobiology & Nutritional Sciences, Institute for Genomic Biology, University of Illinois Christopher Counter, Ph.D. Dept. of Oncology and Cancer Biology, Duke University Medical Center Eric Forsberg, Ph.D Vice President of Business Development, Infigen Merete Fredholm, D.V.M., Ph.D., Dr. Vet. Sci., Royal Veterinary and Agricultural Univ.Inst. Animal Science & Animal Health Medicine Thalachallour Mohanakumar, Ph.D. Dept. of Surgery, Pathology and Immunology, Washington University School of Medicine Randall Prather, Ph.D. Department of Reproductive Biotechnology, University of Missouri Steven Niemi, D.V.M. Center for Comparative Medicine, Massachusetts General Hospital Hiroshi Yasue, Ph.D. National Institute of Agrobiological Sciences, Sukuba, Japan University of Illinois Hosting Committee: Jonathan Beever, Animal Sciences Sharon Donovan, Food Science & Human Nutrition H. Rex Gaskins, Animal Sciences Russell Jamison, Materials Science & Engineering Lawrence Schook, Animal Sciences Kelley Tappenden, Food Science & Human Nutrition Michael Tumbleson, Agricultural Engineering (honorary chair) Matthew Wheeler, Animal Sciences Federico Zuckermann, Veterinary Pathobiology Poster Session Moderators: Bioengineering, Russ Jamison, UIUC Immunology & Infectious Diseases, Federico Zuckermann, UIUC Transplantation (allo & xeno), Doug Smith, Baylor & Mark Rutherford, U.Minn. Nutrition (Obesity and Diabetes), Sharon Donovan, UIUC Genomics and Cloning, Jon Beever, UIUC & Max Rothschild, ISU Cardiovascular, Rex Gaskins, UIUC Physiology, Jack Odle, NCSU Cancer, Craig Beattie, UNR Clinical Models, Steve Niemi, Harvard Sponsored by: Institute for Genomic Biology, College of Agricultural, Consumer, and Environmental Services, College of Liberal Arts & Sciences, College of Veterinary Medicine, and Office of the Vice-Chancellor of Research at the University of Illinois at Urbana-Champaign National Institutes for Health (Grant no. -
Regulates Target Gene Transcription Via Single-Stranded DNA Response Elements
TLS/FUS (translocated in liposarcoma/fused in sarcoma) regulates target gene transcription via single-stranded DNA response elements Adelene Y. Tan1, Todd R. Riley, Tristan Coady, Harmen J. Bussemaker, and James L. Manley2 Department of Biological Sciences, Columbia University, New York, NY 10027 Contributed by James L. Manley, February 29, 2012 (sent for review December 9, 2011) TLS/FUS (TLS) is a multifunctional protein implicated in a wide range TLS has also been linked to splicing. It contains an RNP-type of cellular processes, including transcription and mRNA processing, RNA-binding domain and associates directly with SR protein as well as in both cancer and neurological disease. However, little is splicing factors (11). TET proteins have been detected in spliceo- currently known about TLS target genes and how they are recog- somes (12), and TLS was found associated with RNAP II and nized. Here, we used ChIP and promoter microarrays to identify snRNPs in a transcription and splicing complex in vitro (13). It is genes potentially regulated by TLS. Among these genes, we detected unclear whether and how TLS recruits splicing factors to sites of a number that correlate with previously known functions of TLS, active transcription, but one possibility is through its interaction and confirmed TLS occupancy at several of them by ChIP. We also with TBP and the TFIID complex. detected changes in mRNA levels of these target genes in cells where Here we provide insight into TLS regulation of RNAP II-tran- scribed genes. We used ChIP followed by promoter microarray TLS levels were altered, indicative of both activation and repression. -
Functional Analysis of Structural Variation in the 2D and 3D Human Genome
FUNCTIONAL ANALYSIS OF STRUCTURAL VARIATION IN THE 2D AND 3D HUMAN GENOME by Conor Mitchell Liam Nodzak A dissertation submitted to the faculty of The University of North Carolina at Charlotte in partial fulfillment of the requirements for the degree of Doctor of Philosophy in Bioinformatics and Computational Biology Charlotte 2019 Approved by: Dr. Xinghua Mindy Shi Dr. Rebekah Rogers Dr. Jun-tao Guo Dr. Adam Reitzel ii c 2019 Conor Mitchell Liam Nodzak ALL RIGHTS RESERVED iii ABSTRACT CONOR MITCHELL LIAM NODZAK. Functional analysis of structural variation in the 2D and 3D human genome. (Under the direction of DR. XINGHUA MINDY SHI) The human genome consists of over 3 billion nucleotides that have an average distance of 3.4 Angstroms between each base, which equates to over two meters of DNA contained within the 125 µm3 volume diploid cell nuclei. The dense compaction of chromatin by the supercoiling of DNA forms distinct architectural modules called topologically associated domains (TADs), which keep protein-coding genes, noncoding RNAs and epigenetic regulatory elements in close nuclear space. It has recently been shown that these conserved chromatin structures may contribute to tissue-specific gene expression through the encapsulation of genes and cis-regulatory elements, and mutations that affect TADs can lead to developmental disorders and some forms of cancer. At the population-level, genomic structural variation contributes more to cumulative genetic difference than any other class of mutation, yet much remains to be studied as to how structural variation affects TADs. Here, we study the func- tional effects of structural variants (SVs) through the analysis of chromatin topology and gene activity for three trio families sampled from genetically diverse popula- tions from the Human Genome Structural Variation Consortium. -
Open Data for Differential Network Analysis in Glioma
International Journal of Molecular Sciences Article Open Data for Differential Network Analysis in Glioma , Claire Jean-Quartier * y , Fleur Jeanquartier y and Andreas Holzinger Holzinger Group HCI-KDD, Institute for Medical Informatics, Statistics and Documentation, Medical University Graz, Auenbruggerplatz 2/V, 8036 Graz, Austria; [email protected] (F.J.); [email protected] (A.H.) * Correspondence: [email protected] These authors contributed equally to this work. y Received: 27 October 2019; Accepted: 3 January 2020; Published: 15 January 2020 Abstract: The complexity of cancer diseases demands bioinformatic techniques and translational research based on big data and personalized medicine. Open data enables researchers to accelerate cancer studies, save resources and foster collaboration. Several tools and programming approaches are available for analyzing data, including annotation, clustering, comparison and extrapolation, merging, enrichment, functional association and statistics. We exploit openly available data via cancer gene expression analysis, we apply refinement as well as enrichment analysis via gene ontology and conclude with graph-based visualization of involved protein interaction networks as a basis for signaling. The different databases allowed for the construction of huge networks or specified ones consisting of high-confidence interactions only. Several genes associated to glioma were isolated via a network analysis from top hub nodes as well as from an outlier analysis. The latter approach highlights a mitogen-activated protein kinase next to a member of histondeacetylases and a protein phosphatase as genes uncommonly associated with glioma. Cluster analysis from top hub nodes lists several identified glioma-associated gene products to function within protein complexes, including epidermal growth factors as well as cell cycle proteins or RAS proto-oncogenes. -
Genome-Wide DNA Methylation Dynamics During Epigenetic
Gómez‑Redondo et al. Clin Epigenet (2021) 13:27 https://doi.org/10.1186/s13148‑021‑01003‑x RESEARCH Open Access Genome‑wide DNA methylation dynamics during epigenetic reprogramming in the porcine germline Isabel Gómez‑Redondo1*† , Benjamín Planells1†, Sebastián Cánovas2,3, Elena Ivanova4, Gavin Kelsey4,5 and Alfonso Gutiérrez‑Adán1 Abstract Background: Prior work in mice has shown that some retrotransposed elements remain substantially methylated during DNA methylation reprogramming of germ cells. In the pig, however, information about this process is scarce. The present study was designed to examine the methylation profles of porcine germ cells during the time course of epigenetic reprogramming. Results: Sows were artifcially inseminated, and their fetuses were collected 28, 32, 36, 39, and 42 days later. At each time point, genital ridges were dissected from the mesonephros and germ cells were isolated through magnetic‑ activated cell sorting using an anti‑SSEA‑1 antibody, and recovered germ cells were subjected to whole‑genome bisulphite sequencing. Methylation levels were quantifed using SeqMonk software by performing an unbiased analysis, and persistently methylated regions (PMRs) in each sex were determined to extract those regions showing 50% or more methylation. Most genomic elements underwent a dramatic loss of methylation from day 28 to day 36, when the lowest levels were shown. By day 42, there was evidence for the initiation of genomic re‑methylation. We identifed a total of 1456 and 1122 PMRs in male and female germ cells, respectively, and large numbers of transpos‑ able elements (SINEs, LINEs, and LTRs) were found to be located within these PMRs. Twenty‑one percent of the introns located in these PMRs were found to be the frst introns of a gene, suggesting their regulatory role in the expression of these genes. -
Download Article As
Published December 3, 2012 JCB: Article KIF14 negatively regulates Rap1a–Radil signaling during breast cancer progression Syed M. Ahmed,1 Brigitte L. Thériault,4 Maruti Uppalapati,2 Catherine W.N. Chiu,1 Brenda L. Gallie,4 Sachdev S. Sidhu,2 and Stéphane Angers1,3 1Department of Pharmaceutical Sciences, Leslie Dan Faculty of Pharmacy, 2Terrence Donnelly Center for Cellular and Biomolecular Research, and 3Department of Biochemistry, Faculty of Medicine, University of Toronto, Toronto, Ontario M5S 1A1, Canada 4Campbell Family Cancer Research Institute, Ontario Cancer Institute, University Health Network, Toronto, Ontario M5G 2M9, Canada he small GTPase Rap1 regulates inside-out integrin tethering Radil on microtubules. The depletion of KIF14 activation and thereby influences cell adhesion, mi- led to increased cell spreading, altered focal adhesion dy- T gration, and polarity. Several Rap1 effectors have namics, and inhibition of cell migration and invasion. We been described to mediate the cellular effects of Rap1 in a also show that Radil is important for breast cancer cell context-dependent manner. Radil is emerging as an im- proliferation and for metastasis in mice. Our findings pro- Downloaded from portant Rap effector implicated in cell spreading and vide evidence that the concurrent up-regulation of Rap1 migration, but the molecular mechanisms underlying its activity and increased KIF14 levels in several cancers is functions are unclear. We report here that the kinesin KIF14 needed to reach optimal levels of Rap1–Radil signaling, associates with the PDZ domain of Radil and negatively integrin activation, and cell–matrix adhesiveness required regulates Rap1-mediated inside-out integrin activation by for tumor progression. -
KIF14 Negatively Regulates Rap1a–Radil Signaling During Breast Cancer Progression
JCB: Article KIF14 negatively regulates Rap1a–Radil signaling during breast cancer progression Syed M. Ahmed,1 Brigitte L. Thériault,4 Maruti Uppalapati,2 Catherine W.N. Chiu,1 Brenda L. Gallie,4 Sachdev S. Sidhu,2 and Stéphane Angers1,3 1Department of Pharmaceutical Sciences, Leslie Dan Faculty of Pharmacy, 2Terrence Donnelly Center for Cellular and Biomolecular Research, and 3Department of Biochemistry, Faculty of Medicine, University of Toronto, Toronto, Ontario M5S 1A1, Canada 4Campbell Family Cancer Research Institute, Ontario Cancer Institute, University Health Network, Toronto, Ontario M5G 2M9, Canada he small GTPase Rap1 regulates inside-out integrin tethering Radil on microtubules. The depletion of KIF14 activation and thereby influences cell adhesion, mi- led to increased cell spreading, altered focal adhesion dy- T gration, and polarity. Several Rap1 effectors have namics, and inhibition of cell migration and invasion. We been described to mediate the cellular effects of Rap1 in a also show that Radil is important for breast cancer cell context-dependent manner. Radil is emerging as an im- proliferation and for metastasis in mice. Our findings pro- portant Rap effector implicated in cell spreading and vide evidence that the concurrent up-regulation of Rap1 migration, but the molecular mechanisms underlying its activity and increased KIF14 levels in several cancers is functions are unclear. We report here that the kinesin KIF14 needed to reach optimal levels of Rap1–Radil signaling, associates with the PDZ domain of Radil and negatively integrin activation, and cell–matrix adhesiveness required regulates Rap1-mediated inside-out integrin activation by for tumor progression. Introduction The small G-protein Rap1 is an important mediator of integrin Rap1a-mediated inside-out activation of integrins, adhesion, and inside-out signaling, which plays a pivotal role in adhesion, spreading of human fibrosarcoma cells (Ahmed et al., 2010). -
Karyotypic and Molecular Genetic Changes Associated with Fetal Cardiovascular Abnormalities: Results of a Retrospective 4-Year Ultrasonic Diagnosis Study
Int. J. Biol. Sci. 2013, Vol. 9 463 Ivyspring International Publisher International Journal of Biological Sciences 2013; 9(5):463-471. doi: 10.7150/ijbs.5404 Research Paper Karyotypic and Molecular Genetic Changes Associated With Fetal Cardiovascular Abnormalities: Results of a Retrospective 4-Year Ultrasonic Diagnosis Study Bihui Bao1,2,*, Yu Wang2,*, Hua Hu1, Hong Yao1, Yuyan Li1, Shuai Tang1, Lihong Zheng2, Yan Xu1, Zhiqing Liang1, 1. Department of Gynecology and Obstetrics, Southwest Hospital, Third Military Medical University, Chongqing 400038, China; 2. Department of Gynecology and Obstetrics, Chengdu Military General Hospital, Chengdu 610083, China. * These authors equally contributed to this study. Corresponding author: Zhiqing Liang, Department of Gynecology and Obstetrics, Southwest Hospital, Third Military Medical Univer- sity, Chongqing 400038, China. Tel: +86-23-68754409; Fax: 86-23-65461867 E-mail: [email protected]. © Ivyspring International Publisher. This is an open-access article distributed under the terms of the Creative Commons License (http://creativecommons.org/ licenses/by-nc-nd/3.0/). Reproduction is permitted for personal, noncommercial use, provided that the article is in whole, unmodified, and properly cited. Received: 2012.10.17; Accepted: 2013.04.22; Published: 2013.05.09 Abstract Objective: To investigate the incidence of aneuploidy in fetuses with congenital heart defects (CHDs) and to further identify submicroscopic changes and global DNA methylation levels as potential biomarkers in complex CHD cases. Methods: Fetuses at high risk for birth defects or with obvious sonographic anomalies were recruited at the Prenatal Diagnosis Center and Ultrasonic Diagnosis Center. Elective fetal kary- otyping and DNA copy number and promoter methylation analyses were carried out following parental consent. -
Content Based Search in Gene Expression Databases and a Meta-Analysis of Host Responses to Infection
Content Based Search in Gene Expression Databases and a Meta-analysis of Host Responses to Infection A Thesis Submitted to the Faculty of Drexel University by Francis X. Bell in partial fulfillment of the requirements for the degree of Doctor of Philosophy November 2015 c Copyright 2015 Francis X. Bell. All Rights Reserved. ii Acknowledgments I would like to acknowledge and thank my advisor, Dr. Ahmet Sacan. Without his advice, support, and patience I would not have been able to accomplish all that I have. I would also like to thank my committee members and the Biomed Faculty that have guided me. I would like to give a special thanks for the members of the bioinformatics lab, in particular the members of the Sacan lab: Rehman Qureshi, Daisy Heng Yang, April Chunyu Zhao, and Yiqian Zhou. Thank you for creating a pleasant and friendly environment in the lab. I give the members of my family my sincerest gratitude for all that they have done for me. I cannot begin to repay my parents for their sacrifices. I am eternally grateful for everything they have done. The support of my sisters and their encouragement gave me the strength to persevere to the end. iii Table of Contents LIST OF TABLES.......................................................................... vii LIST OF FIGURES ........................................................................ xiv ABSTRACT ................................................................................ xvii 1. A BRIEF INTRODUCTION TO GENE EXPRESSION............................. 1 1.1 Central Dogma of Molecular Biology........................................... 1 1.1.1 Basic Transfers .......................................................... 1 1.1.2 Uncommon Transfers ................................................... 3 1.2 Gene Expression ................................................................. 4 1.2.1 Estimating Gene Expression ............................................ 4 1.2.2 DNA Microarrays ...................................................... -
Comparing the Effects of Vitamin E Tocotrienol-Rich Fraction Supplementation and Α-Tocopherol Supplementation on Gene Expression in Healthy Older Adults
ORIGINAL ARTICLE Comparing the effects of vitamin E tocotrienol-rich fraction supplementation and α-tocopherol supplementation on gene expression in healthy older adults Siti Madiani Abdul Ghani,I Jo Aan Goon,I,* Nor Helwa Ezzah Nor Azman,I Siti Nor Asyikin Zakaria,I Zalina Hamid,II Wan Zurinah Wan NgahI I Department of Biochemistry, Faculty of Medicine, Universiti Kebangsaan Malaysia, Kuala Lumpur, Malaysia. II Sime Darby Foods & Beverages Marketing Sdn Bhd, Petaling Jaya, Selangor, Malaysia. Abdul Ghani SM, Goon JA, Nor Azman NHE, Asyikin Zakaria SN, Hamid Z, Wan Ngah WZ. Comparing the effects of Vitamin E Tocotrienol-Rich Fraction supplementation and α-Tocopherol supplementation on gene expression in healthy older adults. Clinics. 2019;74:e688 *Corresponding author. E-mail: [email protected] OBJECTIVES: This study aims to compare the differential gene expression resulting from tocotrienol-rich fraction and α-tocopherol supplementation in healthy older adults. METHODS: A total of 71 eligible subjects aged 50 to 55 years from Gombak and Kuala Lumpur, Malaysia, were divided into three groups and supplemented with placebo (n=23), α-tocopherol (n=24) or tocotrienol-rich fraction (n=24). Blood samples were collected at baseline and at 3 and 6 months of supplementation for microarray analysis. RESULTS: The number of genes altered by α-tocopherol was higher after 6 months (1,410) than after 3 months (273) of supplementation. α-Tocopherol altered the expression of more genes in males (952) than in females (731). Similarly, tocotrienol-rich fraction modulated the expression of more genes after 6 months (1,084) than after 3 months (596) and affected more genes in males (899) than in females (781). -
Pre-Adolescence DNA Methylation Is Associated
Wang et al. Clin Epigenet (2021) 13:64 https://doi.org/10.1186/s13148-021-01042-4 RESEARCH Open Access Pre-adolescence DNA methylation is associated with BMI status change from pre- to post-adolescence Jiajing Wang1, Hongmei Zhang1* , Faisal I. Rezwan2, Caroline Relton5,6, S. Hasan Arshad3,7 and John W. Holloway4,7 Abstract Background: Previous studies have shown that DNA methylation (DNAm) is associated with body mass index (BMI). However, it is unknown whether DNAm at pre-adolescence is associated with BMI status transition from pre- to post- adolescence. In the Isle of Wight (IoW) birth cohort, genome-wide DNA methylation in whole blood was measured using Illumina Infnium Human450 and EPIC BeadChip arrays in n 325 subjects, and pre- to post-adolescence BMI transition was classifed into four groups: (1) normal to normal, (2) =normal to overweight or obese, (3) overweight or obese to normal, and (4) persistent overweight or obese. We used recursive random forest to screen genome-wide Cytosine-phosphate-Guanine (CpG) sites with DNAm potentially associated with BMI transition for each gender, and the association of BMI status transition with DNAm at an earlier age was assessed via logistic regressions. To evaluate gender specifcity, interactions between DNAm and gender were included in the model. Findings in the IoW cohort were further tested in an independent cohort, the Avon Longitudinal Study of Parents and Children (ALSPAC). Results: In total, 174 candidate CpGs were selected including CpGs from screening and CpGs previously associated correctionally with BMI in children and adults. Of these 174 CpGs, pre-adolescent DNAm of 38 CpGs in the IoW cohort was associated with BMI status transition, including 30 CpGs showing gender-specifc associations. -
Clinical and Molecular Characteristics of MEF2D Fusion-Positive B-Cell
Acute Lymphoblastic Leukemia SUPPLEMENTARY APPENDIX Clinical and molecular characteristics of MEF2D fusion-positive B-cell precursor acute lymphoblastic leukemia in childhood, including a novel translocation resulting in MEF2D-HNRNPH1 gene fusion Kentaro Ohki, 1 Nobutaka Kiyokawa, 1 Yuya Saito, 1,2 Shinsuke Hirabayashi, 1,3 Kazuhiko Nakabayashi, 4 Hitoshi Ichikawa, 5 Yukihide Momozawa, 6 Kohji Okamura, 7 Ai Yoshimi, 1,8 Hiroko Ogata-Kawata, 4 Hiromi Sakamoto, 5 Motohiro Kato, 1 Keitaro Fukushima, 9 Daisuke Hasegawa, 3 Hiroko Fukushima, 10 Masako Imai, 11 Ryosuke Kajiwara, 12 Takashi Koike, 13 Isao Komori, 14 Atsushi Matsui, 15 Makiko Mori, 16 Koichi Moriwaki, 17 Yasushi Noguchi, 18 Myoung-ja Park, 19 Takahiro Ueda, 20 Shohei Yamamoto, 21 Koichi Matsuda, 22 Teruhiko Yoshida, 5 Kenji Matsumoto, 23 Kenichiro Hata, 4 Michiaki Kubo, 6 Yoichi Matsubara, 24 Hiroyuki Takahashi, 25 Takashi Fukushima, 26 Yasuhide Hayashi, 27 Katsuyoshi Koh, 16 Atsushi Man - abe 3 and Akira Ohara 25 for the Tokyo Children’s Cancer Study Group (TCCSG) 1Department of Pediatric Hematology and Oncology Research, National Research Institute for Child Health and Development, Seta - gaya-ku, Tokyo; 2Department of Hematology/Oncology, Tokyo Metropolitan Children’s Medical Center, Fuchu-shi; 3Department of Pedi - atrics, St. Luke's International Hospital, Chuo-ku, Tokyo; 4Department of Maternal-Fetal Biology, National Research Institute for Child Health and Development, Setagaya-ku, Tokyo; 5Fundamental Innovative Oncology Core, National Cancer Center Research Institute,