Ground Shock Effects from Accidental Explosions
Total Page:16
File Type:pdf, Size:1020Kb
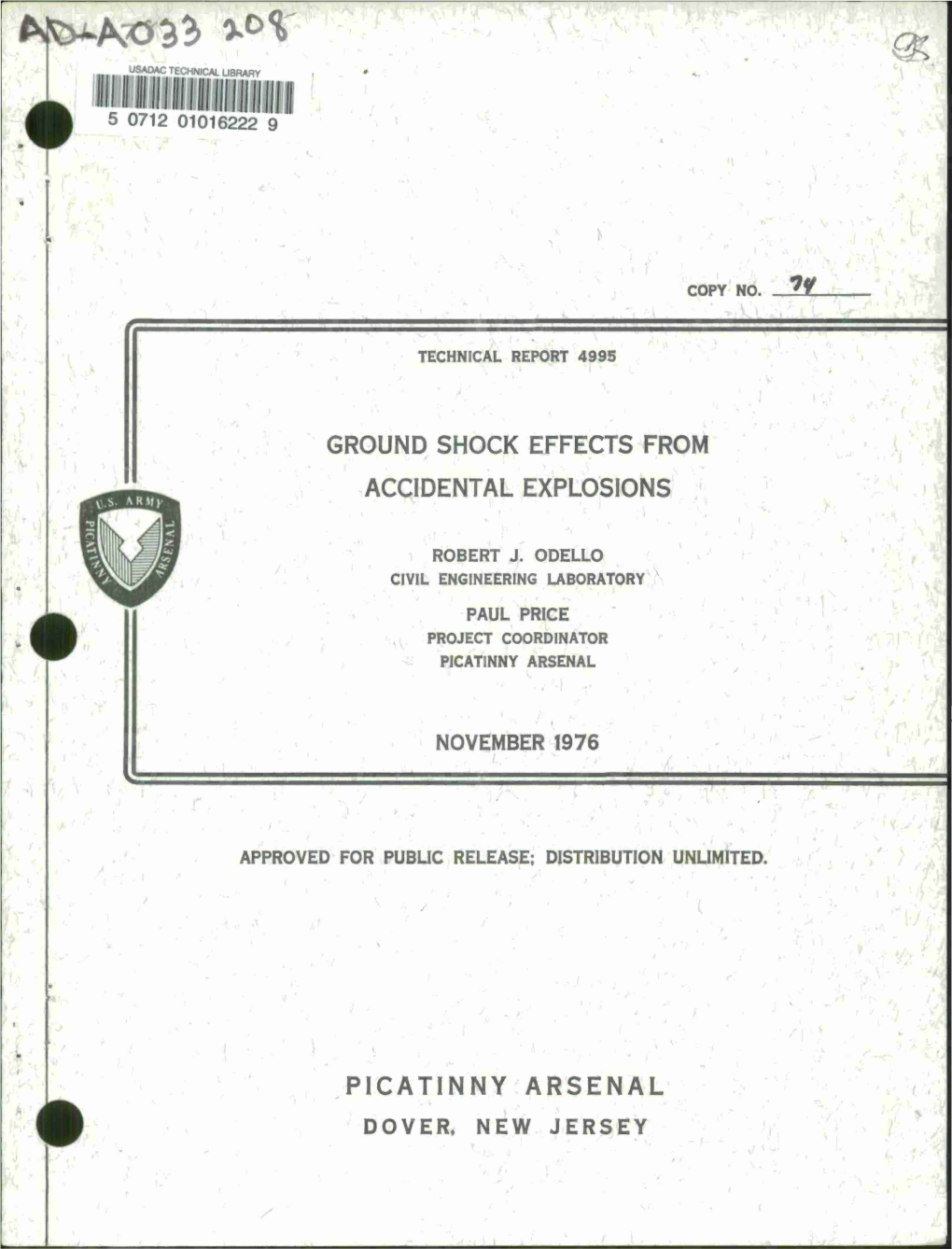
Load more
Recommended publications
-
I Llllll L111 Llill Lllll Lllll Llill Ll1111
NASA SP-5077 T I llllll lllll111 llill lllll lllll llill llll1111 A REPORT BY R. E. Keith Prepared under contract for NASA by Battelle Memorial Institute Techaoiogy Utilizatioti Divisioiz OFFICE OF TECHNOLOGY UTILIZATION 1969 NATIONAL AERONAUTICS AND SPACE ADMINISTRATION WaJhington, D.C. NOTICE * This document was prepared under the sponsorship of the National Aeronautics and Space Administration. Neither the United States Government nor any person acting on behalf of the United States Government assumes any liability resulting from the use of the information contained in this document, or warrants that such use will be free from privately owned rights. For Sale by the Superintendent of Documents, US. Government Printing Office, Washington,D.C. 20402 Price 35 cents Library of Congress Catalog Card Number 77-603553 To assure the greatest possible benefits to the public from the na- tion's space program, the engineers and scientists at NASA research centers and contractors continuously screen the emerging technology for innovative ideas, concepts, working processes, methods, tools, etc. Many of these have applications in non-aerospace business and in- dustry. This is one of a series of publications issued by NASA aimed at the goal of transferring space-derived technology to other spheres of activity. This report reviews major developments in the technology of en- capsulation, potting, and embedment of electronic modules, and the materials used in these processes. Because the literature in this field is voluminous, the coverage oi material already well summarized in previous reports and studies has been avoided. A list of major books, bibliographies, and reviews on the subject is provided. -
Agency (DOD) Program Charts Feb 2019
Index to Charts: Guide to Defense Basic Research Funding Chart #s Topic 1 - 2 Contents 3 - 22 Overview Perspectives, including suggestions on working with DOD Program Officers 23 - 38 By Academic Disciplines 39 - 45 Air Force Office of Scientific Research (AFOSR) 46 - 54 Army Research Office (ARO) 55 Army Corp of Engineers 56 - 59 Army Medical Research and Materials Command (AMRMC) 60 Army Research Institute for Behavioral and Social Science (ARI) 61 - 74 Office of Naval Research (ONR) 75 - 76 Naval Post-Graduate School (NPSG) 77 - 109 Defense Advanced Research Projects Agency (DARPA) 110 - 113 Defense Threat Reduction Agency (DTRA) 114 High Performance Computing 115 MINERVA (social science) 116 - 119 University Research Initiative (URI, including MURI, DURIP, NDSEG) 120 - 122 Defense Medical Research and Development Program (DMRDP) 123 - 130 Congressionally Directed Medical Research Program (CDMRP) 131 US Department of Veterans Affairs 132 University Affiliated Research Centers 133 - 139 Young Investigator / Early Career 140 - 141 Vannevar Bush Faculty Fellow (was NSSEFF) 142 DOD I-Corps 143 Defense Sciences Study Group (DSSG) 144 STEM Education - SMART !1 Revised Jul 2019 Index to Charts: Guidance to Defense Selected Applied Research and Exploratory Development Funding Chart #s Topic 145- 149 Applied Research and Advanced Technology Development 150 - 154 Air Force Materiel Command 155 Air Force Academy 156 - 166 Army Materiel Command 167 US Army Corps of Engineers (USACE) 168 - 172 Naval Research - Applied Research and Adv Technol 173 -
The Burning Ground. However, Operations at the Incinerator Cannot Begin Until the Results of the Trial Burn Are Accepted by the NJDEP
Picatinnyis an Official Hawk Watch Site PICATINNY RECORD OF DECISION SITE 34 - THE BURNING GROUND FINAL JANUARY 2005 TABLE OF CONTENTS Section Page 1.0 DECLARATION 1-1 1.1 SITE NAME AND LOCATION 1-1 1.2 STATEMENT OF BASIS AND PURPOSE 1-1 1.3 ASSESSMENT OF THE SITE 1-2 1.4 DESCRIPTION OF THE SELECTED REMEDY: CAPPING WITH AN IMPERMEABLE MODIFIED ASPHALT PAVEMENT, LAND-USE RESTRICTIONS, AND ONGOING MONITORING 1-2 1.5 STATUTORY DETERMINATIONS 1-6 1.6 ROD DATA CERTIFICATION CHECKLIST 1-6 1.7 AUTHORIZING SIGNATURE 1-6 2.0 DECISION SUMMARY 2-1 2.1 SITE NAME, LOCATION, AND DESCRIPTION 2-1 2.2 SITE HISTORY AND ENFORCEMENT ACTIVITIES 2-1 2.2.1 Picatinny Background 2-1 2.2.2 Site 34 Background 2-1 2.2.3 Enforcement Activities 2-2 2.3 COMMUNITY PARTICIPATION 2-4 2.4 SCOPE AND ROLE OF RESPONSE ACTION 2-4 2.5 SITE CHARACTERISTICS 2-5 2.5.1 Conceptual Site Model 2-5 2.5.2 Physical Characteristics 2-6 2.5.3 Summary and Findings of Site Investigations 2-9 2.6 CURRENT AND POTENTIAL FUTURE LAND USES 2-10 2.7 SUMMARY OF SITE RISKS 2-10 2.7.1 Human Health Risk Assessment 2-11 2.7.2 Ecological Risk Assessment 2-15 2.8 REMEDIAL ACTION OBJECTIVES 2-18 2.9 DESCRIPTION OF ALTERNATIVES 2-18 2.9.1 Alternative 1: No Action 2-19 2.9.2 Alternative 2: Institutional Controls, including Land-Use Restrictions, and Ongoing Groundwater Monitoring/Use Restrictions 2-19 2.9.3 Alternative 3: Capping with an Impermeable Soil and Synthetic Multilayer Cap, Land-Use Restrictions, and Ongoing Groundwater Monitoring/Use Restrictions 2-21 2.9.4 Alternative 4: Capping with an Impermeable -
Automated Canopy and Payload Motion Estimation Using Vision Based Methods
AIAA 2015-2171 Aerodynamic Decelerator Systems Technology Conferences 30 Mar - 2 Apr 2015, Daytona Beach, FL 23rd AIAA Aerodynamic Decelerator Systems Technology Conference Automated Canopy and Payload Motion Estimation Using Vision Based Methods Ryan J. Decker,* US Army Armament Research Development and Engineering Center, Picatinny Arsenal, NJ 07806 Oleg A. Yakimenko† Naval Postgraduate School, Monterey, CA 93943-5107 This this paper advocates the use of automated computer vision and image processing techniques for characterization of parachute cargo systems. It considers two applications in which test video can be used to extract useful information about the behavior of these systems. The first application deals with identifying the relative motion of the decelerator canopy compared to that of the payload using a simple upward facing camera mounted on the top of the payload. The second application employs similar algorithms, but uses multiple ground-based cameras to reveal a six degree of freedom trajectory histories of parachute and parafoil-payload systems. Abbreviations 2D/3D = two / three dimensional αsp = spatial angle of attack θsp = spatial pitch angle ADS = aerodynamic decelerator system {c} = camera/image coordinate frame CAD = computer aided design CCD = charge-coupled device CMDP = Common Mission Debrief Program COTS = commercial off-the-shelf FFT = fast Fourier transform GPS = global positioning system IMU = inertial measurement unit MEMS = micro electromechanical systems P = position (xyz) PADS = precision air-drop system RGB = red-green-blue conventional color digital image TSPI = time space position information SMD = surface mount device V = velocity (xyz) YPG = Yuma Proving Ground, AZ Downloaded by NAVAL POSTGRADUATE SCHOOL on March 16, 2016 | http://arc.aiaa.org DOI: 10.2514/6.2015-2171 I. -
REPORT 2D Session HOUSE of REPRESENTATIVES 105–470 "!
105TH CONGRESS REPORT 2d Session HOUSE OF REPRESENTATIVES 105±470 "! MAKING SUPPLEMENTAL APPROPRIATIONS AND RESCIS- SIONS FOR THE FISCAL YEAR ENDING SEPTEMBER 30, 1998, AND FOR OTHER PURPOSES MARCH 27, 1998.ÐCommitted to the Committee of the Whole House on the State of the Union and ordered to be printed Mr. LIVINGSTON, from the Committee on Appropriations, submitted the following REPORT togehter with DISSENTING VIEWS [To accompany H.R. 3580] The Committee on Appropriations submits the following report in explanation of the accompanying bill making supplemental appro- priations and rescissions for the fiscal year ending September 30, 1998, and for other purposes. BILL HIGHLIGHTS The bill recommended by the Committee includes $17,900,000,000 for the International Monetary Fund. This amount is made up of $3,400,000,000 for loans to the International Mone- tary Fund and $14,500,000,000 for the United States quota, Inter- national Monetary Fund. The bill also includes $505,000,000 for payment of arrearages to the United Nations. This amount is made up of $475,000,000 of advance fiscal year 1999 appropriations and $30,000,000 of advanced fiscal year 2000 appropriations. Also in- cluded in the bill is a mandatory appropriation of $550,000,000 for veterans compensation and pensions. Finally, the bill includes $134,003,000 for regular non-defense discretionary appropriations which are fully offset. ★47±444 2 TITLE IÐSUPPLEMENTAL APPROPRIATIONS CHAPTER 1 DEPARTMENT OF AGRICULTURE OFFICE OF THE SECRETARY The Secretary of Agriculture currently has the authority to com- pensate individuals and other persons for economic losses associ- ated with the presence of karnal bunt in wheat. -
Information on US Use of Land Mines
United States General Accounting Office Report to the Honorable Lane Evans, GAO House of Representatives September 2002 MILITARY OPERATIONS Information on U.S. Use of Land Mines in the Persian Gulf War GAO-02-1003 Contents Letter 1 Results in Brief 2 Background 4 Effect of the Use of Self-Destruct U.S. Land Mines in the Gulf War Is Unknown 6 Extent of U.S. Casualties from Land Mines and Unexploded Ordnance 11 DOD Reports Express Fratricide and Mobility Concerns Relating to the Safety of, and Lack of Knowledge about, Land Mines and Dudfields 20 Agency Comments and Our Evaluation 36 Appendix I Current U.S. Land Mine Inventory 39 Appendix II U.S. Land Mines Available for Use in the Gulf War 44 Appendix III U.S. Gulf War Casualties by Service 51 Appendix IV DOD-Reported Actions That Relate to Land Mine and UXO Concerns 52 Developing Antipersonnel Land-Mine Alternatives and More Capable and Safer Self-Destruct Land Mines 52 Revising Doctrine and Procedures to Better Address Hazardous Submunition Dudfields 57 Increasing Ammunition Reliability and Reducing Dud Rates 60 Appendix V Scope and Methodology 63 Appendix VI Comments from the Department of Defense 67 Page i GAO-02-1003 U.S. Use of Land Mines in the Persian Gulf War Tables Table 1: U.S. Land Mines Reportedly Used in the Gulf War 9 Table 2: Total U.S. Gulf War Casualties 12 Table 3: Descriptions of Casualty Categories 14 Table 4: U.S. Gulf War Casualties from Explosions and All Other Causes 15 Table 5: U.S. -
Naval Warfare Studies Institute (NWSI) Wargaming Center
Naval Postgraduate School Naval Warfare Studies Institute (NWSI) Wargaming Center Quarterly Report - Fall 2020 (1Q FY21) Jeff Appleget, Operations Research, and Rob Burks, Defense Analysis, Directors Naval Warfare Studies Institute: We are proud and excited to direct the NWSI Wargaming Center. To learn more about the NWSI, please see the attached NWSI Slicksheet. How-to Book on the Craft of Wargaming Hits the Streets By Javier Chagoya Naval Postgraduate School (NPS) scholars in the field of wargaming have authored a seminal work that lays out a detailed planning guide for defense planners and analysts in a new book, “The Craft of Wargaming.” The book is co-authored by NPS Senior Lecturer retired Army Col. Jeff Appleget, NPS Associate Professor retired Army Col. Robert Burks, and internationally-recognized Operations Research Analyst Fred Cameron. These three authorities bring more than 100 years of wargaming knowledge to an ever-expanding cadre of wargaming professionals. The book is designed to support defense planners and analysts on their journey from wargaming apprentice to journeyman, with topics of particular interest to commercial wargamers. With its focus on design and development, the book serves a primer for initiates to senior commanders seeking to advance their knowledge and understanding of the wargaming field. For the full article, see: https://www.navy.mil/Press-Office/News-Stories/display-news/Article/2360929/naval- postgraduate-school-publishes-how-to-book-on-wargaming/ Click to hear the authors discuss the motivation behind the book in a podcast with the Naval Institute Press: https://www.usni.org/the-proceedings-podcast/episode-193-craft-of-wargaming The following CIMSEC article discusses the role wargaming should have in DoD education: REVAMPING WARGAMING EDUCATION FOR THE U.S. -
U.S. Army Weapons-Related Directed Energy (DE) Programs: Background and Potential Issues for Congress
U.S. Army Weapons-Related Directed Energy (DE) Programs: Background and Potential Issues for Congress Andrew Feickert Specialist in Military Ground Forces February 12, 2018 Congressional Research Service 7-5700 www.crs.gov R45098 U.S. Army Weapons-Related Directed Energy (DE) Programs Summary The U.S. military has a long and complicated history in developing directed energy (DE) weapons. Many past efforts have failed for a variety of reasons and not all failures were attributed to scientific or technological challenges associated with weaponizing DE. At present, a number of U.S. military DE weapons-related programs are beginning to show promise, such as the Navy’s Laser Weapon System (LaWs), the first ever Department of Defense (DOD) laser weapon to be deployed and approved for operational use, according to the Navy. With a number of U.S. Army weapons-related DE programs showing promise during concept demonstrations and their potential relevance in addressing a number of current and emerging threats to U.S. ground forces, some believe the Army is making progress to field viable DE weapon systems designed to counter rockets, artillery, and mortars (C-RAM) and address certain types of short-range air defense (SHORAD) threats. While DE weapons offer a variety of advantages over conventional kinetic weapons including precision, low cost per shot, and scalable effects, there are also some basic constraints, such as beam attenuation, limited range, and an inability to be employed against non-line-of-sight targets, that will need to be addressed in order to make these weapons effective across the entire spectrum of combat operations. -
A Comparative Analysis of Contemporary 155 Mm Artillery
The current issue and full text archive of this journal is available on Emerald Insight at: www.emeraldinsight.com/2399-6439.htm Contemporary A comparative analysis of 155 mm contemporary 155 mm artillery artillery projectiles projectiles Matilda R. Brady 171 Field Artillery School, United States Army, Fort Sill, Oklahoma, USA, and Received 30 May 2019 Paul Goethals Revised 3 October 2019 4 October 2019 Department of Mathematical Sciences, United States Military Academy, Accepted 4 October 2019 West Point, New York, USA Abstract Purpose – To recover the growing deficit between American and near-peer mobile artillery ranges, the US Army is exploring the use of the M982 Excalibur munition, a family of long-range precision projectiles. This paper aims to analyze the effectiveness of the M982 in comparison to the M795 and M549A1 projectiles to further the understanding of what this new asset contributes. Design/methodology/approach – Based upon doctrinal scenarios for target destruction, a statistical analysis is performed using Monte Carlo simulation to identify a likely probability of kill ratio for the M982. A values-based hierarchical modeling approach is then used to differentiate the M982 from similar-type projectiles quantitatively in terms of several different attributes. Finally, sensitivity analyzes are presented for each of the value attributes, to identify areas where measures may lack robustness in precision. Findings – Based upon a set of seven value measures, such as maximum range, effective range, the expected number of rounds to destroy a target, and the unit cost of a munition, the M982 1a-2 was found to be best suited for engaging point and small area targets. -
The Picatinny Voice Vol
Part 2: SLINGING PICatINNY’s arMaMENts FOR HIstOrY STEM PAGE 5 PAGES 6-7 FIREPOWER AWARDS PAGE 10 The Picatinny Voice Vol. 30 No. 15 https://www.pica.army.mil/evoice Published in the interest of the Picatinny Arsenal, N.J., community November 10, 2017 Picatinny breaks ground on $8.4 million co-generation energy facility Picatinny Arsenal Public Affairs mission processes. “We are exceptionally excited and look- The senior-most official on Army “This $8.4 million, ing forward to the timely construction of installations helped to break ground 2-megawatt co-genera- this facility and we are looking forward to on an $8.4 million energy co-gen- tion facility is one project competing for our eighth Secretary of the eration plant at a ceremony here on of seven, totaling near $17 Army award for energy efficiency.” Nov. 7. million,” said Ivey, Pica- The co-generation design of the facility Participating in the ceremony tinny Arsenal Garrison improves energy efficiency by using natural with Acting Assistant Secretary of Commander. gas to power an electricity generator and to the Army Installation Energy and “Each project is energy generate steam on site. Environment, Jordon Gillis, were efficient, will bring energy The steam is produced from the genera- Picatinny’s Senior Commander, savings, and make our tor’s waste heat, and it is utilized in an exist- Brig. Gen. Alfred Abramson; Gar- installation more energy ing distribution system. Picatinny’s mission rison Commander, Lt. Col. Jeffrey independent by reducing processes have a year-round need for steam Ivey, and Senior Vice President of our dependency on outside energy. -
SMA) Conference
DRAFT UNCLASSIFIED th 12 Annual Strategic Multi-Layer Assessment (SMA) Conference Biographies of Panel Members, Moderators, and Speakers UNCLASSIFIED DRAFT UNCLASSIFIED KEYNOTE SPEAKER General Paul J. Selva Gen. Paul J. Selva serves as the 10th Vice Chairman of the Joint Chiefs of Staff. In this capacity, he is a member of the Joint Chiefs of Staff and the nation’s second highest-ranking military officer. General Selva graduated from the U.S. Air Force Academy in 1980, and completed undergraduate pilot training at Reese AFB, Texas. He has held numerous staff positions and has commanded at the squadron, group, wing and headquarter levels. Prior to his current assignment General Selva was the commander of U.S. Transportation Command, Scott AFB, Illinois. General Selva is a command pilot with more than 3,100 hours in the C-5, C-17A, C-141B, C-37, KC-10, KC-135A and T-37. EDUCATION 1980 Bachelor of Science in Aeronautical Engineering, U.S. Air Force Academy, Colorado Springs, Colo. 1983 Squadron Officer School, Maxwell AFB, Ala. 1984 Master of Science in Management and Human Relations, Abilene Christian University, Abilene, Texas 1992 Air Command and Staff College, Maxwell AFB, Ala., distinguished graduate 1992 Master of Science in Political Science, Auburn University, Montgomery, Ala. 1996 National Defense Fellow, Secretary of Defense Strategic Studies Group, Rosslyn, Va. ASSIGNMENTS 1. June 1980 - July 1981, student, undergraduate pilot training, Reese AFB, Texas 2. July 1981 - December 1984, co-pilot and aircraft commander, 917th Air Refueling Squadron, Dyess AFB, Texas 3. January 1984 - December 1988, co-pilot, aircraft commander, instructor pilot, and flight commander, 32nd Air Refueling Squadron, Barksdale AFB, La. -
AGENDA 0730 REGISTRATION/CONTINENTAL BREAKFAST Sponsored by Kaman Fuzing Tuesday, 10 June
ARMAMENTS TECHNOLOGY FIRE POWER FORUM AGENDA 0730 REGISTRATION/CONTINENTAL BREAKFAST Sponsored by Kaman Fuzing TUESdaY, 10 JUne 0830 WELCOME: Andy McHugh—Vice-Chairman of the Board MG Paul Greenberg, USA (Ret)—PSA Executive Director & Event Chair 0840 OPENING REMARKS: Brigadier General William Phillips, USA—Commander, Joint Munitions and Lethality Life Cycle Management Command and Program Executive Officer for Ammunition, Picatinny Arsenal, NJ 0900 KEYNOTE ADDRESS: Major General David W. Eidsaune, USAF—Commander, Air Armament Center, and the Air Force Program Executive Officer for Weapons, Air Force Materiel Command, Eglin Air Force Base, FL 0945 NETWORKING REFRESHMENT BREAK Sponsored by Whitney, Bradley & Brown, Inc. 1015 THE EVOLUTION OF ARTILLERY FOR INCREASED EFFECTIVENESS: Dominick De Mella—Chief Cannon Artillery Division, ARDEC, Picatinny Arsenal, NJ 1045 JOINT IED DEFEAT ORGANIZATION (JIEDDO) REQUIREMENTS: Colonel Ray Nelson, USA—United States Military Academy, West Point, NY 1115 ARDEC's Role in Countering the IED Threat: Ray Carr— ARDEC Counter Terrorism Technology Team Lead, U.S. Army, ARDEC, Picatinny Arsenal, NJ 1145 LUNCHEON Sponsored by Lockheed Martin Company 1315 U.S. ARMY JOINT MUNITIONS COMMAND ACTIVITIES: Jyuji Hewitt—Deputy to Commander, Joint Munitions Command, Rock Island, IL 1345 PICATINNY LABORATORY INITIATIVES: Dr. Joe Lannon—Director US Army ARDEC, Picatinny Arsenal, NJ 1415 HIGHLY PRECISE MUNITIONS OVERVIEW: Charles Kelly—Staff Specialist, Land Warfare & Munitions, OUSD (AT&L) 1500 NETWORKING REFRESHMENT BREAK