Edinburgh Research Explorer
Total Page:16
File Type:pdf, Size:1020Kb
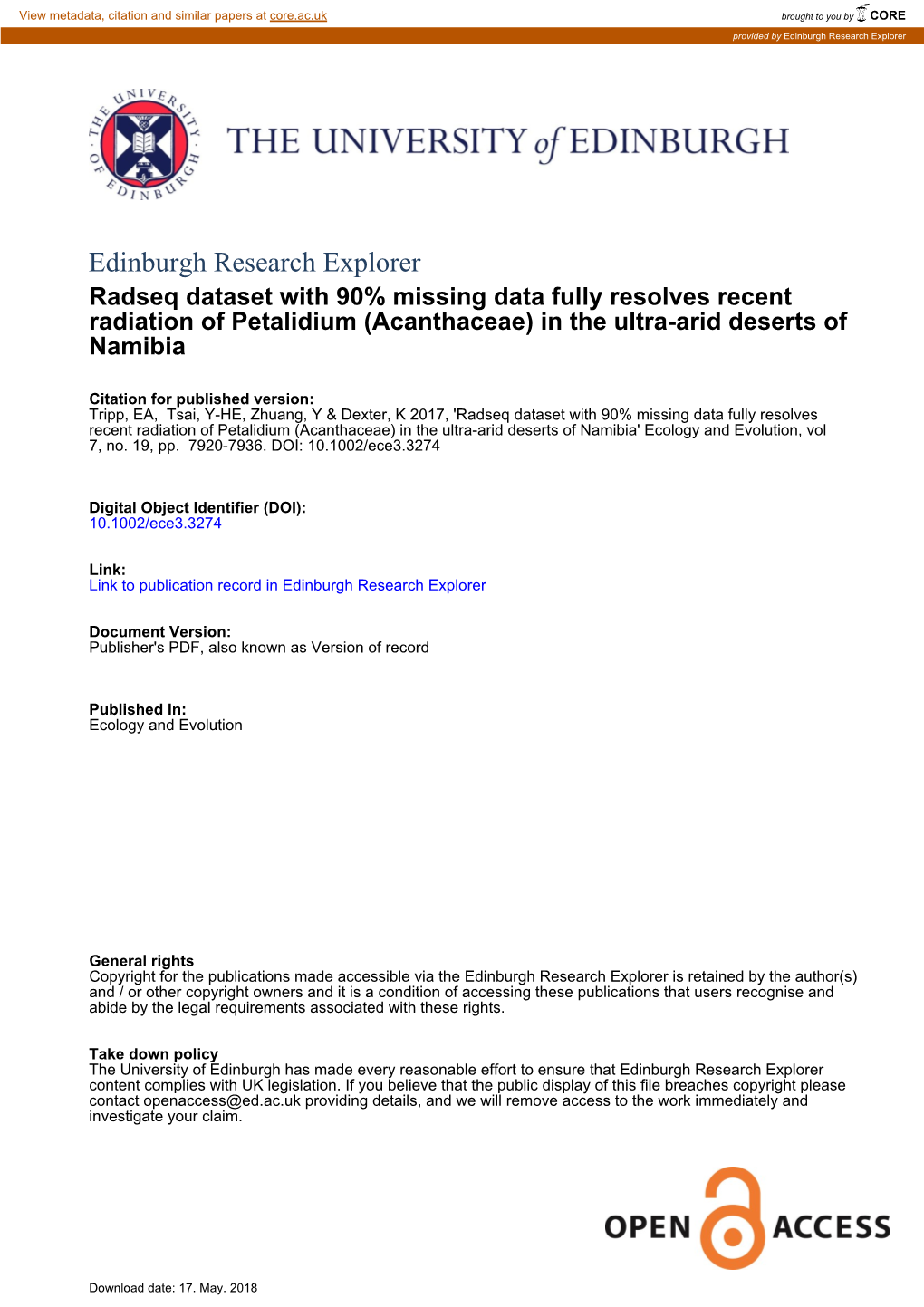
Load more
Recommended publications
-
Phylogeny of Dyschoriste (Acanthaceae) Noravit Chumchim Rancho Santa Ana Botanic Garden, Claremont, California
Aliso: A Journal of Systematic and Evolutionary Botany Volume 33 | Issue 2 Article 2 2015 Phylogeny of Dyschoriste (Acanthaceae) Noravit Chumchim Rancho Santa Ana Botanic Garden, Claremont, California Lucinda A. McDade Rancho Santa Ana Botanic Garden, Claremont, California Amanda E. Fisher Rancho Santa Ana Botanic Garden, Claremont, California Follow this and additional works at: http://scholarship.claremont.edu/aliso Part of the Botany Commons, and the Evolution Commons Recommended Citation Chumchim, Noravit; McDade, Lucinda A.; and Fisher, Amanda E. (2015) "Phylogeny of Dyschoriste (Acanthaceae)," Aliso: A Journal of Systematic and Evolutionary Botany: Vol. 33: Iss. 2, Article 2. Available at: http://scholarship.claremont.edu/aliso/vol33/iss2/2 Aliso, 33(2), pp. 77–89 ISSN 0065-6275 (print), 2327-2929 (online) PHYLOGENY OF DYSCHORISTE (ACANTHACEAE) NORAVIT CHUMCHIM,1,3 LUCINDA A. MCDADE,1 AND AMANDA E. FISHER1,2 1Rancho Santa Ana Botanic Garden and Claremont Graduate University, 1500 North College Avenue, Claremont, California 91711; 2Department of Biological Sciences, California State University, Long Beach, 1250 Bellflower Boulevard, Long Beach, California 90840 3Corresponding author ([email protected]) ABSTRACT The pantropical and poorly known genus Dyschoriste (Acanthaceae) is sister to Strobilanthopsis within subtribe Petalidiinae. The present study included 38 accessions of 28 species as sources of DNA data for one nuclear (nrITS) and four chloroplast (intergenic spacers: psbA-trnH, trnS-trnG, ndhF-rpl32, rpl32- trnL(uag)) regions to provide an estimate of the phylogeny of the genus. We found that Dyschoriste is strongly supported as monophyletic inclusive of Apassalus, Chaetacanthus, and Sautiera. Within Dyschoriste, three geographically cohesive lineages were recovered with moderate to strong support: a mainland African clade, a Caribbean and southeastern United States clade, and a South and Central America clade. -
Vegetation Survey of Mount Gorongosa
VEGETATION SURVEY OF MOUNT GORONGOSA Tom Müller, Anthony Mapaura, Bart Wursten, Christopher Chapano, Petra Ballings & Robin Wild 2008 (published 2012) Occasional Publications in Biodiversity No. 23 VEGETATION SURVEY OF MOUNT GORONGOSA Tom Müller, Anthony Mapaura, Bart Wursten, Christopher Chapano, Petra Ballings & Robin Wild 2008 (published 2012) Occasional Publications in Biodiversity No. 23 Biodiversity Foundation for Africa P.O. Box FM730, Famona, Bulawayo, Zimbabwe Vegetation Survey of Mt Gorongosa, page 2 SUMMARY Mount Gorongosa is a large inselberg almost 700 sq. km in extent in central Mozambique. With a vertical relief of between 900 and 1400 m above the surrounding plain, the highest point is at 1863 m. The mountain consists of a Lower Zone (mainly below 1100 m altitude) containing settlements and over which the natural vegetation cover has been strongly modified by people, and an Upper Zone in which much of the natural vegetation is still well preserved. Both zones are very important to the hydrology of surrounding areas. Immediately adjacent to the mountain lies Gorongosa National Park, one of Mozambique's main conservation areas. A key issue in recent years has been whether and how to incorporate the upper parts of Mount Gorongosa above 700 m altitude into the existing National Park, which is primarily lowland. [These areas were eventually incorporated into the National Park in 2010.] In recent years the unique biodiversity and scenic beauty of Mount Gorongosa have come under severe threat from the destruction of natural vegetation. This is particularly acute as regards moist evergreen forest, the loss of which has accelerated to alarming proportions. -
Phylogenomic Study of Monechma Reveals Two Divergent Plant Lineages of Ecological Importance in the African Savanna and Succulent Biomes
diversity Article Phylogenomic Study of Monechma Reveals Two Divergent Plant Lineages of Ecological Importance in the African Savanna and Succulent Biomes 1, , 2, 3 4,5 Iain Darbyshire * y, Carrie A. Kiel y, Corine M. Astroth , Kyle G. Dexter , Frances M. Chase 6 and Erin A. Tripp 7,8 1 Royal Botanic Gardens, Kew, Richmond, Surrey TW9 3AE, UK 2 Rancho Santa Ana Botanic Garden, Claremont Graduate University, 1500 North College Avenue, Claremont, CA 91711, USA; [email protected] 3 Scripps College, 1030 Columbia Avenue, Claremont, CA 91711, USA; [email protected] 4 School of GeoSciences, University of Edinburgh, Edinburgh EH9 3JN, UK; [email protected] 5 Royal Botanic Garden Edinburgh, Edinburgh EH3 5LR, UK 6 National Herbarium of Namibia, Ministry of Environment, Forestry and Tourism, National Botanical Research Institute, Private Bag 13306, Windhoek 10005, Namibia; [email protected] 7 Department of Ecology and Evolutionary Biology, University of Colorado, UCB 334, Boulder, CO 80309, USA; [email protected] 8 Museum of Natural History, University of Colorado, UCB 350, Boulder, CO 80309, USA * Correspondence: [email protected]; Tel.: +44-(0)20-8332-5407 These authors contributed equally. y Received: 1 May 2020; Accepted: 5 June 2020; Published: 11 June 2020 Abstract: Monechma Hochst. s.l. (Acanthaceae) is a diverse and ecologically important plant group in sub-Saharan Africa, well represented in the fire-prone savanna biome and with a striking radiation into the non-fire-prone succulent biome in the Namib Desert. We used RADseq to reconstruct evolutionary relationships within Monechma s.l. and found it to be non-monophyletic and composed of two distinct clades: Group I comprises eight species resolved within the Harnieria clade, whilst Group II comprises 35 species related to the Diclipterinae clade. -
Lamiales – Synoptical Classification Vers
Lamiales – Synoptical classification vers. 2.6.2 (in prog.) Updated: 12 April, 2016 A Synoptical Classification of the Lamiales Version 2.6.2 (This is a working document) Compiled by Richard Olmstead With the help of: D. Albach, P. Beardsley, D. Bedigian, B. Bremer, P. Cantino, J. Chau, J. L. Clark, B. Drew, P. Garnock- Jones, S. Grose (Heydler), R. Harley, H.-D. Ihlenfeldt, B. Li, L. Lohmann, S. Mathews, L. McDade, K. Müller, E. Norman, N. O’Leary, B. Oxelman, J. Reveal, R. Scotland, J. Smith, D. Tank, E. Tripp, S. Wagstaff, E. Wallander, A. Weber, A. Wolfe, A. Wortley, N. Young, M. Zjhra, and many others [estimated 25 families, 1041 genera, and ca. 21,878 species in Lamiales] The goal of this project is to produce a working infraordinal classification of the Lamiales to genus with information on distribution and species richness. All recognized taxa will be clades; adherence to Linnaean ranks is optional. Synonymy is very incomplete (comprehensive synonymy is not a goal of the project, but could be incorporated). Although I anticipate producing a publishable version of this classification at a future date, my near- term goal is to produce a web-accessible version, which will be available to the public and which will be updated regularly through input from systematists familiar with taxa within the Lamiales. For further information on the project and to provide information for future versions, please contact R. Olmstead via email at [email protected], or by regular mail at: Department of Biology, Box 355325, University of Washington, Seattle WA 98195, USA. -
A Synoptical Classification of the Lamiales
Lamiales – Synoptical classification vers. 2.0 (in prog.) Updated: 13 December, 2005 A Synoptical Classification of the Lamiales Version 2.0 (in progress) Compiled by Richard Olmstead With the help of: D. Albach, B. Bremer, P. Cantino, C. dePamphilis, P. Garnock-Jones, R. Harley, L. McDade, E. Norman, B. Oxelman, J. Reveal, R. Scotland, J. Smith, E. Wallander, A. Weber, A. Wolfe, N. Young, M. Zjhra, and others [estimated # species in Lamiales = 22,000] The goal of this project is to produce a working infraordinal classification of the Lamiales to genus with information on distribution and species richness. All recognized taxa will be clades; adherence to Linnaean ranks is optional. Synonymy is very incomplete (comprehensive synonymy is not a goal of the project, but could be incorporated). Although I anticipate producing a publishable version of this classification at a future date, my near-term goal is to produce a web-accessible version, which will be available to the public and which will be updated regularly through input from systematists familiar with taxa within the Lamiales. For further information on the project and to provide information for future versions, please contact R. Olmstead via email at [email protected], or by regular mail at: Department of Biology, Box 355325, University of Washington, Seattle WA 98195, USA. Lamiales – Synoptical classification vers. 2.0 (in prog.) Updated: 13 December, 2005 Acanthaceae (~201/3510) Durande, Notions Elém. Bot.: 265. 1782, nom. cons. – Synopsis compiled by R. Scotland & K. Vollesen (Kew Bull. 55: 513-589. 2000); probably should include Avicenniaceae. Nelsonioideae (7/ ) Lindl. ex Pfeiff., Nomencl. -
And Phylogenetic Significance Long Been Recognised (Bachmann, 1886
BLUMEA 24 (1978) 101-117 Epidermalhairs of Acanthaceae Khwaja+J. Ahmad Plant Anatomy Laboratory, National Botanic Gardens, Lucknow-226001, India. Summary two belonging to Structure and distribution of the foliar epidermal hairs of 109 species and varieties 39 hairs have Acanthaceae have been studied. Both and non-glandularepidermal genera ofthe family glandular hairs The sub- been recorded in the investigated taxa. The glandular may be subsessile or long-stalked. Glandular head of Glandular head 2-celled, and ii) sessile glandular hairs are two types: i) panduriform, —8- more-celled. Subfamilies Nelsonioideae and Thunbergioideae are character- globular or disc-shaped, 2 or hairs i«*H hv thfi nanduriform hairs, while Mendoncioideae and Acanthoideae have glandular with a globular also only in nine species. Non-glandular hairs are widely head. Long-stalked glandular hairs are present or multicellular are in all but ten They be unicellular, distributed in the family; they present species. may the hairs are of at species uniseriate; rarely they are branched. Though non-glandular diagnosticimportance The like Barleria, and Äphelandra, are quite characteristic. present level only, in some genera Ruttya,, they delimitation of the family Acanthaceae, involving the transfer study does not support Bremekamp's (1965) and the of his subfamilies Thunber- Nelsonioideae to raising of Lindau's (1895) subfamily Scrophulariaceae, rank of families. the retention of Nelsonioideae, gioideae and Mendoncioideae to the independent Instead, Acanthaceae is favoured. Thunbergioideae, Mendoncioideae, and Acanthoideae within the family Introduction The taxonomic and phylogenetic significance of trichomes has long been recognised number ofworkers Solereder, 2 Cowan, by a (Bachmann, 1886; 1908; Cooper, 193 ! 195°! According to Carlquist Metcalfe & Chalk, 1950; Goodspeed, 1954; and Sporne, 1956). -
Using the Checklist N W C
Using the checklist • The arrangement of the checklist is alphabetical by family followed by genus, grouped under Pteridophyta, Gymnosperms, Monocotyledons and Dicotyledons. • All species and synonyms are arranged alphabetically under genus. • Accepted names are in bold print while synonyms or previously-used names are in italics. • In the case of synonyms, the currently used name follows the equals sign (=), and only refers to usage in Zimbabwe. • Distribution information is included under the current name. • The letters N, W, C, E, and S, following each listed taxon, indicate the known distribution of species within Zimbabwe as reflected by specimens in SRGH or cited in the literature. Where the distribution is unknown, we have inserted Distr.? after the taxon name. • All species known or suspected to be fully naturalised in Zimbabwe are included in the list. They are preceded by an asterisk (*). Species only known from planted or garden specimens were not included. Mozambique Zambia Kariba Mt. Darwin Lake Kariba N Victoria Falls Harare C Nyanga Mts. W Mutare Gweru E Bulawayo GREAT DYKEMasvingo Plumtree S Chimanimani Mts. Botswana N Beit Bridge South Africa The floristic regions of Zimbabwe: Central, East, North, South, West. A checklist of Zimbabwean vascular plants A checklist of Zimbabwean vascular plants edited by Anthony Mapaura & Jonathan Timberlake Southern African Botanical Diversity Network Report No. 33 • 2004 • Recommended citation format MAPAURA, A. & TIMBERLAKE, J. (eds). 2004. A checklist of Zimbabwean vascular plants. -
Time-Calibrated Phylogenies of Hummingbirds and Hummingbird-Pollinated Plants Reject a Hypothesis of Diffuse Co-Evolution Erin A
Aliso: A Journal of Systematic and Evolutionary Botany Volume 31 | Issue 2 Article 5 2013 Time-Calibrated Phylogenies of Hummingbirds and Hummingbird-Pollinated Plants Reject a Hypothesis of Diffuse Co-Evolution Erin A. Tripp Department of Ecology and Evolutionary Biology, University of Colorado, Boulder Lucinda A. McDade Rancho Santa Ana Botanic Garden, Claremont, California Follow this and additional works at: http://scholarship.claremont.edu/aliso Recommended Citation Tripp, Erin A. and McDade, Lucinda A. (2013) "Time-Calibrated Phylogenies of Hummingbirds and Hummingbird-Pollinated Plants Reject a Hypothesis of Diffuse Co-Evolution," Aliso: A Journal of Systematic and Evolutionary Botany: Vol. 31: Iss. 2, Article 5. Available at: http://scholarship.claremont.edu/aliso/vol31/iss2/5 Aliso, 31(2), pp. 89–103 ’ 2013, The Author(s), CC-BY-NC TIME-CALIBRATED PHYLOGENIES OF HUMMINGBIRDS AND HUMMINGBIRD-POLLINATED PLANTS REJECT A HYPOTHESIS OF DIFFUSE CO-EVOLUTION ERIN A. TRIPP1,3 AND LUCINDA A. MCDADE2 1University of Colorado, Boulder, Museum of Natural History and Department of Ecology and Evolutionary Biology, UCB 334, Boulder, Colorado 80309 2Rancho Santa Ana Botanic Garden, 1500 North College Avenue, Claremont, California 91711 3Corresponding author ([email protected]) ABSTRACT Neotropical ecosystems house levels of species diversity that are unmatched by any other region on Earth. One hypothesis to explain this celebrated diversity invokes a model of biotic interactions in which interspecific interactions drive diversification of two (or more) lineages. When the impact of the interaction on diversification is reciprocal, diversification of the lineages should be contemporaneous. Although past studies have provided evidence needed to test alternative models of diversification such as those involving abiotic factors (e.g., Andean uplift, shifting climatological regimes), tests of the biotic model have been stymied by lack of evolutionary time scale for symbiotic partners. -
Flowering Plants of Africa 65 (2017) a B Flowering Plants of Africa 65 (2017) Flowering Plants of Africa
Flowering Plants of Africa 65 (2017) a b Flowering Plants of Africa 65 (2017) Flowering Plants of Africa A peer-reviewed journal containing colour plates with descrip- tions of flowering plants of Africa and neighbouring islands Edited by Alicia Grobler with assistance of Gillian Condy Volume 65 Pretoria 2017 ii Flowering Plants of Africa 65 (2017) Editorial board R.R. Klopper South African National Biodiversity Institute, Pretoria, RSA P.C. Zietsman National Museum, Bloemfontein, RSA Referees and other co-workers on this volume R.H. Archer, South African National Biodiversity Institute, Pretoria, RSA S.P. Bester, South African National Biodiversity Institute, Pretoria, RSA G.J. Bredenkamp, Eco-Agent, Pretoria, RSA G. Germishuizen, ex South African National Biodiversity Institute, Pretoria, RSA C.A. González-Martínez, Universidad Nacional Autónoma de México, Mexico City, Mexico A. Grobler, South African National Biodiversity Institute, Pretoria, RSA D. Goyder, Royal Botanic Gardens, Kew, UK L. Henderson, Agricultural Research Council, Pretoria, RSA P.P.J. Herman, South African National Biodiversity Institute, Pretoria, RSA T.P. Jaca, South African National Biodiversity Institute, Pretoria, RSA R.R. Klopper, South African National Biodiversity Institute, Pretoria, RSA M.M. le Roux, South African National Biodiversity Institute, Pretoria, RSA T. Manyelo, South African National Biodiversity Institute, Pretoria, RSA J.J. Meyer, South African National Biodiversity Institute, Pretoria, RSA S.M. Mothogoane, South African National Biodiversity Institute, Pretoria, RSA T. Nkonki, South African National Biodiversity Institute, Pretoria, RSA T.G. Rebelo, South African National Biodiversity Institute, Cape Town, RSA E. Retief, ex South African National Biodiversity Institute, Pretoria, RSA S.J. Siebert, North-West University, Potchefstroom, RSA V. -
Namibia 2010.Pdf
Mar 2010, Namibia Tripp Report This report with photos can be seen at: http://rsabg.org/acanthaceae/index.php?option=com_content&view=article&id=61&Itemid=76 Wednesday, 17 Mar At present, I'm listening to Swedish National Public Radio over the satellite TV, doing DNA alignments, and waiting in my hotel room for the sun to rise (I made the mistake of thinking about coffee, which I hauled from the US, when I awoke at 03:00 under the influence of heavy jetlag). After 35 hours in transit, only 21 of which were in air, I reached Windhoek, Namibia late last night. I am here to collect species of Acanthaceae, as part of a recently launched NSF project on the systematics and evolution of the Ruellieae lineage. Specifically, I am drawn here by one plant group that has undergone a spectacular radiation contained almost wholly within the borders of Namibia: the genus Petalidium. In the field, we will attempt to find and study as many of the ca. 30 species of Petalidium as possible; I am also targeting the genus Ruelliopsis, Ruellia, and several other genera in Acanthaceae. The eventual goal is a worldwide synthesis of evolutionary relationships and diversification in Ruellieae, with relevant taxonomic revisions where necessary. Today I will visit the WIND Herbarium at the National Botanical Research Institute to make contact with the local botanists and then go retrieve the research permit from the Ministry of the Environment and Tourism office. This evening I will be joined by my colleague Dr. Kyle Dexter, based at CNRS (Centre National de la Recherche Scientifique) in Toulouse, France. -
Evolutionary and Ecological Drivers of Plant Flavonoids Across a Large Lat- Itudinal Gradient
Accepted Manuscript Evolutionary and Ecological Drivers of Plant Flavonoids Across A Large Lat- itudinal Gradient Erin A. Tripp, Yongbin Zhuang, Matthew Schreiber, Heather Stone, Andrea E. Berardi PII: S1055-7903(17)30807-2 DOI: https://doi.org/10.1016/j.ympev.2018.07.004 Reference: YMPEV 6230 To appear in: Molecular Phylogenetics and Evolution Received Date: 7 November 2017 Revised Date: 3 July 2018 Accepted Date: 5 July 2018 Please cite this article as: Tripp, E.A., Zhuang, Y., Schreiber, M., Stone, H., Berardi, A.E., Evolutionary and Ecological Drivers of Plant Flavonoids Across A Large Latitudinal Gradient, Molecular Phylogenetics and Evolution (2018), doi: https://doi.org/10.1016/j.ympev.2018.07.004 This is a PDF file of an unedited manuscript that has been accepted for publication. As a service to our customers we are providing this early version of the manuscript. The manuscript will undergo copyediting, typesetting, and review of the resulting proof before it is published in its final form. Please note that during the production process errors may be discovered which could affect the content, and all legal disclaimers that apply to the journal pertain. Evolutionary and Ecological Drivers of Plant Flavonoids Across A Large Latitudinal Gradient Running title: Flavonoids in Ruellia Erin A. Tripp1,2†, Yongbin Zhuang1,2,3†, Matthew Schreiber1,2,4, Heather Stone1,2, and Andrea E. Berardi1,5 †Authors contributed equally 1Department of Ecology and Evolutionary Biology, University of Colorado, UCB 334, Boulder, CO 80309, USA 2Museum of -
Download/List/Vascular.Asp; Accessed Jan
i PHYLOGENY, BIOGEOGRAPHY, AND REPRODUCTIVE BIOLOGY OF THE COSMOPOLITAN FLOWERING PLANT GENUS STELLARIA L. by MATHEW THOMAS SHARPLES B.A., University of Massachusetts, 2008 A dissertation submitted to the Faculty of the Graduate School of the University of Colorado in partial fulfillment of the requirement for the degree of Doctor of Philosophy Department of Ecology and Evolutionary Biology 2019 ii This dissertation entitled: Phylogeny, biogeography, and reproductive biology of the cosmopolitan flowering plant genus Stellaria L. written by Mathew Thomas Sharples has been approved for the Department of Ecology and Evolutionary Biology ________________________________ Dr. Erin A. Tripp ________________________________ Dr. Jeffry Mitton ________________________________ Dr. Mitchell McGlaughlin ________________________________ Dr. Stacey D. Smith ________________________________ Dr. William Bowman Date 4 November 2019 The final copy of this thesis has been examined by the signatories, and we find that both the content and the form meet acceptable presentation standards of scholarly work in the above mentioned discipline. iii Sharples, Mathew Thomas (Ph.D., Ecology and Evolutionary Biology) Phylogeny, biogeography, and reproductive biology of the cosmopolitan flowering plant genus Stellaria L. Dissertation directed by Associate Professor and COLO Herbarium Curator Dr. Erin A. Tripp The flowering plant genus Stellaria L. (Caryophyllaceae; the “starworts”) numbers around 112 species and exhibits a cosmopolitan distribution. To gain familiarity