Inert Gas Dilution Effect on Flammability Limits Of
Total Page:16
File Type:pdf, Size:1020Kb
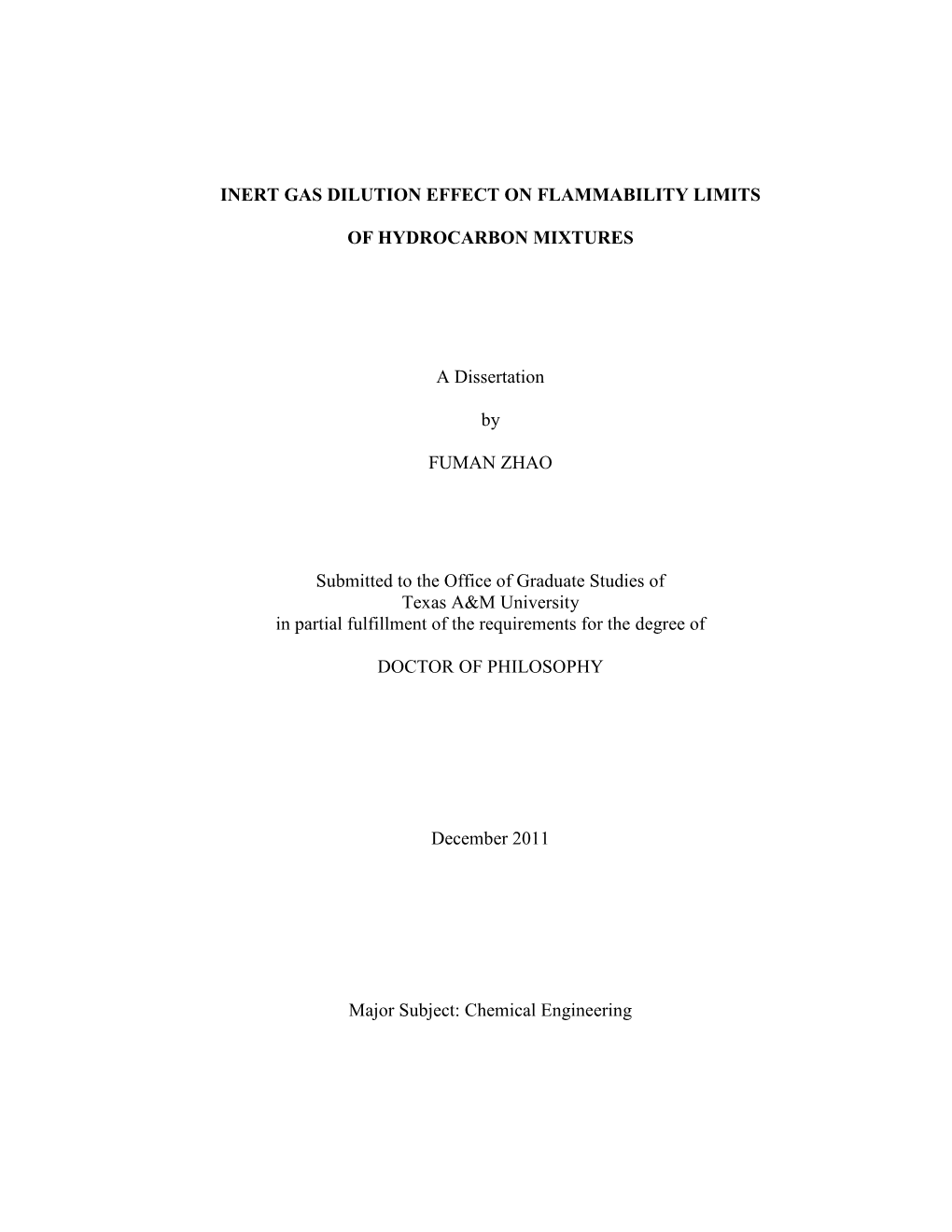
Load more
Recommended publications
-
C9-14 Aliphatic [2-25% Aromatic] Hydrocarbon Solvents Category SIAP
CoCAM 2, 17-19 April 2012 BIAC/ICCA SIDS INITIAL ASSESSMENT PROFILE Chemical C -C Aliphatic [2-25% aromatic] Hydrocarbon Solvents Category Category 9 14 Substance Name CAS Number Stoddard solvent 8052-41-3 Chemical Names Kerosine, petroleum, hydrodesulfurized 64742-81-0 and CAS Naphtha, petroleum, hydrodesulfurized heavy 64742-82-1 Registry Solvent naphtha, petroleum, medium aliphatic 64742-88-7 Numbers Note: Substances in this category are also commonly known as mineral spirits, white spirits, or Stoddard solvent. CAS Number Chemical Description † 8052-41-3 Includes C8 to C14 branched, linear, and cyclic paraffins and aromatics (6 to 18%), <50ppmV benzene † 64742-81-0 Includes C9 to C14 branched, linear, and cyclic paraffins and aromatics (10 to Structural 25%), <100 ppmV benzene Formula † and CAS 64742-82-1 Includes C8 to C13 branched, linear, and cyclic paraffins and aromatics (15 to 25%), <100 ppmV benzene Registry † Numbers 64742-88-7 Includes C8 to C13 branched, linear, and cyclic paraffins and aromatics (14 to 20%), <50 ppmV benzene Individual category member substances are comprised of aliphatic hydrocarbon molecules whose carbon numbers range between C9 and C14; approximately 80% of the aliphatic constituents for a given substance fall within the C9-C14 carbon range and <100 ppmV benzene. In some instances, the carbon range of a test substance is more precisely defined in the test protocol. In these instances, the specific carbon range (e.g. C8-C10, C9-C10, etc.) will be specified in the SIAP. * It should be noted that other substances defined by the same CAS RNs may have boiling ranges outside the range of 143-254° C and that these substances are not covered by the category. -
1 Refinery and Petrochemical Processes
3 1 Refinery and Petrochemical Processes 1.1 Introduction The combination of high demand for electric cars and higher automobile engine effi- ciency in the future will mean less conversion of petroleum into fuels. However, the demand for petrochemicals is forecast to rise due to the increase in world popula- tion. With this, it is expected that modern and more innovative technologies will be developed to serve the growth of the petrochemical market. In a refinery process, petroleum is converted into petroleum intermediate prod- ucts, including gases, light/heavy naphtha, kerosene, diesel, light gas oil, heavy gas oil, and residue. From these intermediate refinery product streams, several fuels such as fuel gas, liquefied petroleum gas, gasoline, jet fuel, kerosene, auto diesel, and other heavy products such as lubricants, bunker oil, asphalt, and coke are obtained. In addition, these petroleum intermediates can be further processed and separated into products for petrochemical applications. In this chapter, petroleum will be introduced first. Petrochemicals will be intro- duced in the second part of the chapter. Petrochemicals – the main subject of this book – will address three major areas, (i) the production of the seven cornerstone petrochemicals: methane and synthesis gas, ethylene, propylene, butene, benzene, toluene, and xylenes; (ii) the uses of the seven cornerstone petrochemicals, and (iii) the technology to separate petrochemicals into individual components. 1.2 Petroleum Petroleum is derived from the Latin words “petra” and “oleum,” which means “rock” and “oil,” respectively. Petroleum also is known as crude oil or fossil fuel. It is a thick, flammable, yellow-to-black mixture of gaseous, liquid, and solid hydrocarbons formed from the remains of plants and animals. -
Dehydrogenation by Heterogeneous Catalysts
Dehydrogenation by Heterogeneous Catalysts Daniel E. Resasco School of Chemical Engineering and Materials Science University of Oklahoma Encyclopedia of Catalysis January, 2000 1. INTRODUCTION Catalytic dehydrogenation of alkanes is an endothermic reaction, which occurs with an increase in the number of moles and can be represented by the expression Alkane ! Olefin + Hydrogen This reaction cannot be carried out thermally because it is highly unfavorable compared to the cracking of the hydrocarbon, since the C-C bond strength (about 246 kJ/mol) is much lower than that of the C-H bond (about 363 kJ/mol). However, in the presence of a suitable catalyst, dehydrogenation can be carried out with minimal C-C bond rupture. The strong C-H bond is a closed-shell σ orbital that can be activated by oxide or metal catalysts. Oxides can activate the C-H bond via hydrogen abstraction because they can form O-H bonds, which can have strengths comparable to that of the C- H bond. By contrast, metals cannot accomplish the hydrogen abstraction because the M- H bonds are much weaker than the C-H bond. However, the sum of the M-H and M-C bond strengths can exceed the C-H bond strength, making the process thermodynamically possible. In this case, the reaction is thought to proceed via a three centered transition state, which can be described as a metal atom inserting into the C-H bond. The C-H bond bridges across the metal atom until it breaks, followed by the formation of the corresponding M-H and M-C bonds.1 Therefore, dehydrogenation of alkanes can be carried out on oxides as well as on metal catalysts. -
Phase Equilibria of Supercritical Carbon Dioxide and Hydrocarbon Mixtures
Louisiana State University LSU Digital Commons LSU Historical Dissertations and Theses Graduate School 1992 Phase Equilibria of Supercritical Carbon Dioxide and Hydrocarbon Mixtures. Hyo-guk Lee Louisiana State University and Agricultural & Mechanical College Follow this and additional works at: https://digitalcommons.lsu.edu/gradschool_disstheses Recommended Citation Lee, Hyo-guk, "Phase Equilibria of Supercritical Carbon Dioxide and Hydrocarbon Mixtures." (1992). LSU Historical Dissertations and Theses. 5325. https://digitalcommons.lsu.edu/gradschool_disstheses/5325 This Dissertation is brought to you for free and open access by the Graduate School at LSU Digital Commons. It has been accepted for inclusion in LSU Historical Dissertations and Theses by an authorized administrator of LSU Digital Commons. For more information, please contact [email protected]. INFORMATION TO USERS This manuscript has been reproduced from the microfilm master. UMI films the text directly from the original or copy submitted. Thus, some thesis and dissertation copies are in typewriter face, while others may be from any type of computer printer. The quality of this reproduction is dependent upon the quality of the copy submitted. Broken or indistinct print, colored or poor quality illustrations and photographs, print bleedthrough, substandard margins, and improper alignment can adversely afreet reproduction. In the unlikely event that the author did not send UMI a complete manuscript and there are missing pages, these will be noted. Also, if unauthorized copyright material had to be removed, a note will indicate the deletion. Oversize materials (e.g., maps, drawings, charts) are reproduced by sectioning the original, beginning at the upper left-hand corner and continuing from left to right in equal sections with small overlaps. -
Thermal Conductivity Correlations for Minor Constituent Fluids in Natural
Fluid Phase Equilibria 227 (2005) 47–55 Thermal conductivity correlations for minor constituent fluids in natural gas: n-octane, n-nonane and n-decaneଝ M.L. Huber∗, R.A. Perkins Physical and Chemical Properties Division, National Institute of Standards and Technology, Boulder, CO 80305, USA Received 21 July 2004; received in revised form 29 October 2004; accepted 29 October 2004 Abstract Natural gas, although predominantly comprised of methane, often contains small amounts of heavier hydrocarbons that contribute to its thermodynamic and transport properties. In this manuscript, we review the current literature and present new correlations for the thermal conductivity of the pure fluids n-octane, n-nonane, and n-decane that are valid over a wide range of fluid states, from the dilute-gas to the dense liquid, and include an enhancement in the critical region. The new correlations represent the thermal conductivity to within the uncertainty of the best experimental data and will be useful for researchers working on thermal conductivity models for natural gas and other hydrocarbon mixtures. © 2004 Elsevier B.V. All rights reserved. Keywords: Alkanes; Decane; Natural gas constituents; Nonane; Octane; Thermal conductivity 1. Introduction 2. Thermal conductivity correlation Natural gas is a mixture of many components. Wide- We represent the thermal conductivity λ of a pure fluid as ranging correlations for the thermal conductivity of the lower a sum of three contributions: alkanes, such as methane, ethane, propane, butane and isobu- λ ρ, T = λ T + λ ρ, T + λ ρ, T tane, have already been developed and are available in the lit- ( ) 0( ) r( ) c( ) (1) erature [1–6]. -
Measurements of Higher Alkanes Using NO Chemical Ionization in PTR-Tof-MS
Atmos. Chem. Phys., 20, 14123–14138, 2020 https://doi.org/10.5194/acp-20-14123-2020 © Author(s) 2020. This work is distributed under the Creative Commons Attribution 4.0 License. Measurements of higher alkanes using NOC chemical ionization in PTR-ToF-MS: important contributions of higher alkanes to secondary organic aerosols in China Chaomin Wang1,2, Bin Yuan1,2, Caihong Wu1,2, Sihang Wang1,2, Jipeng Qi1,2, Baolin Wang3, Zelong Wang1,2, Weiwei Hu4, Wei Chen4, Chenshuo Ye5, Wenjie Wang5, Yele Sun6, Chen Wang3, Shan Huang1,2, Wei Song4, Xinming Wang4, Suxia Yang1,2, Shenyang Zhang1,2, Wanyun Xu7, Nan Ma1,2, Zhanyi Zhang1,2, Bin Jiang1,2, Hang Su8, Yafang Cheng8, Xuemei Wang1,2, and Min Shao1,2 1Institute for Environmental and Climate Research, Jinan University, 511443 Guangzhou, China 2Guangdong-Hongkong-Macau Joint Laboratory of Collaborative Innovation for Environmental Quality, 511443 Guangzhou, China 3School of Environmental Science and Engineering, Qilu University of Technology (Shandong Academy of Sciences), 250353 Jinan, China 4State Key Laboratory of Organic Geochemistry and Guangdong Key Laboratory of Environmental Protection and Resources Utilization, Guangzhou Institute of Geochemistry, Chinese Academy of Sciences, 510640 Guangzhou, China 5State Joint Key Laboratory of Environmental Simulation and Pollution Control, College of Environmental Sciences and Engineering, Peking University, 100871 Beijing, China 6State Key Laboratory of Atmospheric Boundary Physics and Atmospheric Chemistry, Institute of Atmospheric Physics, Chinese -
Development of Health-Based Alternative to the Total Petroleum Hydrocarbon (Tph) Parameter
INTERIM FINAL PETROLEUM REPORT: DEVELOPMENT OF HEALTH-BASED ALTERNATIVE TO THE TOTAL PETROLEUM HYDROCARBON (TPH) PARAMETER Prepared for: Bureau of Waste Site Cleanup Massachusetts Department of Environmental Protection Boston, MA Prepared by: Office of Research and Standards Massachusetts Department of Environmental Protection Boston, MA and ABB Environmental Services, Inc. Wakefield, MA AUGUST 1994 INTERIM FINAL PETROLEUM REPORT: DEVELOPMENT OF HEALTH-BASED ALTERNATIVE TO THE TOTAL PETROLEUM HYDROCARBON (TPH) PARAMETER TABLE OF CONTENTS Section Title Page No. EXECUTIVE SUMMARY .................................................................................................. v AUTHORS AND REVIEWERS .......................................................................................... ix 1.0 INTRODUCTION.......................................................................................................1-1 1.1 Background ...............................................................................................1-1 1.2 Purpose......................................................................................................1-2 1.3 Approach ...................................................................................................1-6 2.0 HAZARD IDENTIFICATION FOR PETROLEUM HYDROCARBONS.....................2-1 2.1 Composition of Petroleum Products...........................................................2-1 2.2 Toxic Effects of Whole Products................................................................2-5 2.2.1 Gasoline.........................................................................................2-5 -
2. Alkanes and Cycloalkanes
BOOKS 1) Organic Chemistry Structure and Function, K. Peter C. Vollhardt, Neil Schore, 6th Edition 2) Organic Chemistry, T. W. Graham Solomons, Craig B. Fryhle 3) Organic Chemistry: A Short Course, H. Hart, L. E. Craine, D. J. Hart, C. M. Hadad, 4) Organic Chemistry: A Brief Course, R. C. Atkins, F.A. Carey Hazırlayanlar : Prof. Dr. Pervin Ünal Civcir ve Doç. Dr. Melike Kalkan 2. ALKANES AND CYCLOALKANES 2.1 Nomenclature of alkanes 2.2 Physical Properties of Alkanes 2.3 Preparation of Alkanes 2.3.1 Hydrogenation of Alkenes and Alkynes 2.3.2 Alkanes From Alkyl Halides 2.3.2.1 Reduction 2.3.2.2 Wurtz Reaction 2.3.2.3 Hydrolysis of Grignard Reagent 2.3.3 Reduction of Carbonyl Compounds 2.3.3.1 Clemmensen Reduction 2.3.3.2 Wolf- Kıschner Reduction 2.3.3.3 Alkanes from Carboxylic Acids 2.4 Reactions of Alkanes 2.4.1 Radicalic substitution reactions 2.4.2 Combustion Reactions 2.4.3 Nitration 2.4.4 Cracking 2.5 Cycloalkanes 2.5.1 Nomenclature of Cycloalkanes 2.5.2 Conformations of Cycloalkanes 2.5.3 Substituted Cycloalkanes 2.5.3.1 Monosubstitued Cycloalkanes 2.5.3.2 Disubstitued Cycloalkanes Hazırlayanlar : Prof. Dr. Pervin Ünal Civcir ve Doç. Dr. Melike Kalkan 2.1 Nomenclature of Alkanes Alkanes with increasing numbers of carbon atoms have names are based on the Latin word for the number of carbon atoms in the chain of each molecule. Nomenclature of Straight Chain Alkanes: n CnH2n+2 n-Alkane n CnH2n+2 n-Alkane n CnH2n+2 n-Alkane 1 CH4 methane 6 C6H14 hexane 11 C11H24 undecane 2 C2H6 ethane 7 C7H16 heptane 12 C12H26 dodecane 3 C3H8 propane 8 C8H18 octane 13 C13H28 tridecane 4 C4H10 butane 9 C9H20 nonane 14 C14H30 tetradecane 5 C5H12 pentane 10 C10H22 decane 15 C15H32 pentadecane 2.2 Physical Properties of Alkanes (i) The first four alkanes are gases at room temperature. -
(C2–C8) Sources and Sinks Around the Arabian Peninsula
Atmos. Chem. Phys., 19, 7209–7232, 2019 https://doi.org/10.5194/acp-19-7209-2019 © Author(s) 2019. This work is distributed under the Creative Commons Attribution 4.0 License. Non-methane hydrocarbon (C2–C8) sources and sinks around the Arabian Peninsula Efstratios Bourtsoukidis1, Lisa Ernle1, John N. Crowley1, Jos Lelieveld1, Jean-Daniel Paris2, Andrea Pozzer1, David Walter3, and Jonathan Williams1 1Department of Atmospheric Chemistry, Max Planck Institute for Chemistry, Mainz 55128, Germany 2Laboratoire des Sciences du Climat et de l’Environnement, CEA-CNRS-UVSQ, UMR8212, IPSL, Gif-sur-Yvette, France 3Department of Multiphase Chemistry, Max Planck Institute for Chemistry, Mainz 55128, Germany Correspondence: Efstratios Bourtsoukidis ([email protected]) Received: 29 January 2019 – Discussion started: 14 February 2019 Revised: 25 April 2019 – Accepted: 29 April 2019 – Published: 29 May 2019 Abstract. Atmospheric non-methane hydrocarbons source-dominated areas of the Arabian Gulf (bAG D 0:16) (NMHCs) have been extensively studied around the and along the northern part of the Red Sea (bRSN D 0:22), but globe due to their importance to atmospheric chemistry stronger dependencies are found in unpolluted regions such and their utility in emission source and chemical sink as the Gulf of Aden (bGA D 0:58) and the Mediterranean Sea identification. This study reports on shipborne NMHC (bMS D 0:48). NMHC oxidative pair analysis indicated that measurements made around the Arabian Peninsula during OH chemistry dominates the oxidation of hydrocarbons in the AQABA (Air Quality and climate change in the Arabian the region, but along the Red Sea and the Arabian Gulf the BAsin) ship campaign. -
BUBBLE FORMATION in SUPERSATURATED HYDROCARBON MIXTURES Petroleum Reservoirs, the Mineral and Water Surfaces with for Each Crystal Averaging About 4.5 Sq Cm
T.P. 3441 BUBBLE FORMATION IN SUPERSATURATED HYDRO CARBON MIXTURES HARVEY T. KENNEDY, A AND M COlLEGE OF TEXAS, COlLEGE STATION, TEX., MEMBER AIME, AND CHARLES R. OLSON, OHIO OIL CO., SHREVEPORT, LA., JUNIOR MEMBER AIME Downloaded from http://onepetro.org/jpt/article-pdf/4/11/271/2238408/spe-232-g.pdf by guest on 29 September 2021 ABSTRACT tude, may be calculated for any rate of pressure decline imposed on the reservoir by production. The bearing of the In many investigations of the performance of petroleum res number and distribution of bubbles on reservoir performance ervoirs the assumption is made that the liquid, if below its is discussed. bubble-point pressure, is at all times in equilibrium with gas_ On the other hand, observations by numerous investigators have indicated that gas-liquid systems including hydrocarbon systems, may exhibit supersaturation to the extent of many INTRODUCTION hundred psi in the laboratory. Up to the present, there has been no reliable data on which to judge the actual extent of A liquid system is supersaturated with gas when the amount supersaturation under conditions approaching those existing of gas dissolved exceeds that corresponding to equilibrium at in petroleum reservoirs. the existing pressure and temperature. The degree of super The work reported here deals with observations and meas saturation may be conveniently expressed as the difference urements on mixtures of methane and kerosene in the presence between the bubble-point of the mixture and the prevailing of silica and calcite crystals. Bubbles were observed to form pressure. Thus, if a mixture having a bubble-point of 1,000 on crystal-hydrocarbon surfaces in preference to the glass psi at a given temperature exists in single liquid phase at hydrocarbon interface or to the body of the liquid. -
Unit – 1- Classification of Crude and Refining Scha3002
SCHOOL OF BIO AND CHEMICAL ENGINEERING DEPARTMENT OF CHEMICAL ENGINEERING UNIT – 1- CLASSIFICATION OF CRUDE AND REFINING SCHA3002 1 UNIT I INTRODUCTION Petroleum, along with oil and coal, is classified as a fossil fuel. Fossil fuels are formed when sea plants and animals die, and the remains become buried under several thousand feet of silt, sand or mud. Fossil fuels take millions of years to form and therefore petroleum is also considered to be a non-renewable energy source. Petroleum is formed by hydrocarbons (a hydrocarbon is a compound made up of carbon and hydrogen) with the addition of certain other substances, primarily sulphur. Petroleum in its natural form when first collected is usually named crude oil, and can be clear, green or black and may be either thin like gasoline or thick like tar. In 1859 Edwin Drake sank the first known oil well, this was in Pennsylvania. Since this time oil and petroleum production figure grew exponentially. Originally the primary use of petroleum was as a lighting fuel, once it had been distilled and turned into kerosene. When Edison opened the world's first electricity generating plant in 1882 the demand for kerosene began to drop. However, by this time Henry Ford had shown the world that the automobile would be the best form of transport for decades to come, and gasoline began to be a product in high demand. World War I was the real catalyst for petroleum production, with more petroleum being produced throughout the war than had ever been produced previously. In modern times petroleum is viewed as a valuable commodity, traded around the world in the same way as gold and diamonds. -
Natural Gas Liquids (Ngls) Other Hydrocarbons* Additives and Oxygenates* * Can Also Be Secondary Products
Leonardo Rocha Souza Workshop on Energy Statistics for ASEAN Countries 21-23 November 2017 Kuala Lumpur, Malaysia http://unstats.un.org/unsd/energy Overview 1. Importance of oil 2. Oil classification 3. Refining processes 4. Compiling/Reporting oil data 5. Concluding remarks 2 Importance of oil World TES Still the largest source of world’s energy supply in 2015 Still fundamental for transportation (92.2% of energy used for transport) Source: IEA KWES 2017 3 Importance of Oil Diversification in areas other than transport Even with the rise of liquid biofuels (biodiesel, bioethanol), oil is still much needed for transport 4 Importance of Oil Pie charts illustrating the cross-section of the previous graph 5 Oil classification With the distinction of primary and secondary energy products, the main primary oil products are: Conventional crude oil Natural Gas Liquids (NGLs) Other hydrocarbons* Additives and oxygenates* * can also be secondary products Although Feedstocks are classified in SIEC together with the primary oil products, they are rather (2ary) oil products that return to the refinery to serve as feedstock. 6 4 Oil 41 410 4100 Conventional crude oil 42 420 4200 Natural gas liquids (NGL) 43 430 4300 Refinery feedstocks 44 440 4400 Additives and oxygenates 45 450 4500 Other hydrocarbons 46 Oil products 461 4610 Refinery gas 462 4620 Ethane 463 4630 Liquefied petroleum gases (LPG) 464 4640 Naphtha 465 Gasolines 4651 Aviation gasoline 4652 Motor gasoline 4653 Gasoline-type jet fuel 466 Kerosenes 4661 Kerosene-type jet fuel