Structural and Biochemical Studies of Ribonucleotide
Total Page:16
File Type:pdf, Size:1020Kb
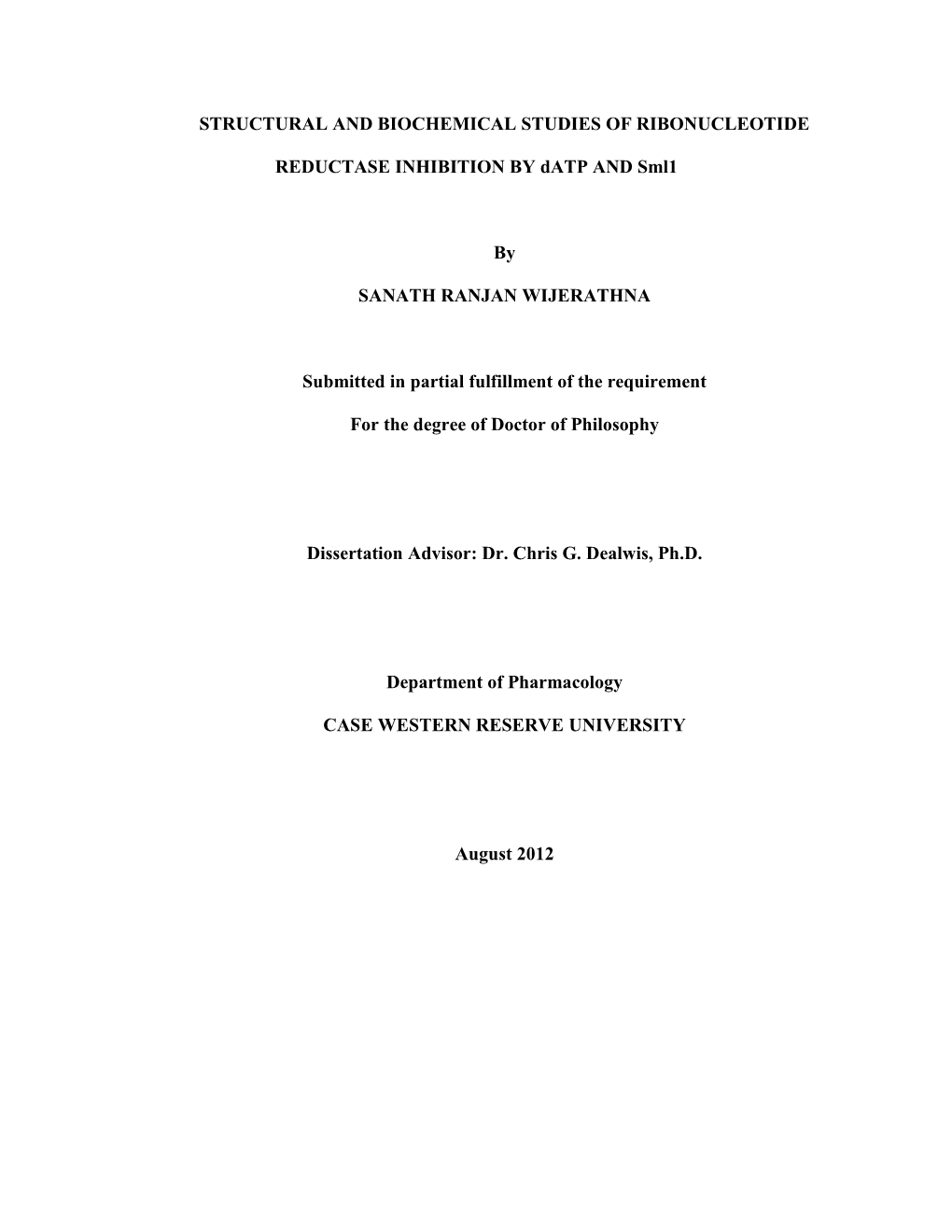
Load more
Recommended publications
-
UMP/CMPK Is Not the Critical Enzyme in the Metabolism of Pyrimidine Ribonucleotide and Activation of Deoxycytidine Analogs in Human RKO Cells
UMP/CMPK Is Not the Critical Enzyme in the Metabolism of Pyrimidine Ribonucleotide and Activation of Deoxycytidine Analogs in Human RKO Cells Rong Hu1, Wing Lam1, Chih-Hung Hsu1,2, Yung-Chi Cheng* 1 Department of Pharmacology, Yale University School of Medicine, New Haven, Connecticut, United States of America, 2 Department of Oncology, National Taiwan University Hospital, Taipei, Taiwan, Republic of China Abstract Background: Human UMP/CMP kinase was identified based on its enzymatic activity in vitro. The role of this protein is considered critical for the maintenance of pyrimidine nucleotide pool profile and for the metabolism of pyrimidine analogs in cells, based on the in vitro study of partially purified enzyme and recombinant protein. However, no detailed study has yet addressed the role of this protein in nucleotide metabolism in cells. Methodology/Principal Findings: Two stable cell lines in which UMP/CMP kinase (mRNA: AF087865, EC 2.7.4.14) can be either up-regulated or down-regulated were developed using Tet-On Gene Expression Systems. The amount and enzymatic activity of UMP/CMP kinase extracted from these two cell lines can be induced up by 500% or down by 95–98%. The ribonucleotides of endogenous pyrimidine as well as the metabolism of exogenous natural pyrimidine nucleosides and their analogs were not susceptible to the altered amount of UMP/CMP kinase in these two stable RKO cell lines. The level of incorporation of pyrimidine nucleoside analogs, such as gemcitabine (dFdC) and troxacitabine (L-OddC), into cellular DNA and their potency in inhibiting cell growth were not significantly altered by up-regulation or down-regulation of UMP/CMP kinase expression in cells. -
Identification and Analysis of Single-Nucleotide Polymorphisms in the Gemcitabine Pharmacologic Pathway
The Pharmacogenomics Journal (2004) 4, 307–314 & 2004 Nature Publishing Group All rights reserved 1470-269X/04 $30.00 www.nature.com/tpj ORIGINAL ARTICLE Identification and analysis of single-nucleotide polymorphisms in the gemcitabine pharmacologic pathway AK Fukunaga1 ABSTRACT 2 Significant variability in the antitumor efficacy and systemic toxicity of S Marsh gemcitabine has been observed in cancer patients. However, there are 1 DJ Murry currently no tools for prospective identification of patients at risk for TD Hurley3 untoward events. This study has identified and validated single-nucleotide HL McLeod2 polymorphisms (SNP) in genes involved in gemcitabine metabolism and transport. Database mining was conducted to identify SNPs in 14 genes 1Department of Clinical Pharmacy and Pharmacy involved in gemcitabine metabolism. Pyrosequencing was utilized to Practice, Purdue University, W. Lafayette, IN, determine the SNP allele frequencies in genomic DNA from European and 2 USA; Departments of Medicine, Genetics, and African populations (n ¼ 190). A total of 14 genetic variants (including 12 Molecular Biology and Pharmacology, Washington University School of Medicine and SNPs) were identified in eight of the gemcitabine metabolic pathway genes. the Siteman Cancer Center, St Louis, MO, USA; The majority of the database variants were observed in population samples. 3Department of Biochemistry and Molecular Nine of the 14 (64%) polymorphisms analyzed have allele frequencies that Biology, Indiana University School of Medicine, were found to be significantly different between the European and African Indianapolis, IN, USA populations (Po0.05). This study provides the first step to identify markers Correspondence: for predicting variability in gemcitabine response and toxicity. Dr HL McLeod, Washington University The Pharmacogenomics Journal (2004) 4, 307–314. -
Genomic Interrogation of Melanoma for Identification of Driver Oncogenic Factors
UC Merced UC Merced Electronic Theses and Dissertations Title Genomic Interrogation of Melanoma for Identification of Driver Oncogenic Factors Permalink https://escholarship.org/uc/item/1fb0q0s2 Author Gupta, Rohit Publication Date 2019 License https://creativecommons.org/licenses/by/4.0/ 4.0 Peer reviewed|Thesis/dissertation eScholarship.org Powered by the California Digital Library University of California UNIVERSITY OF CALIFORNIA, MERCED Genomic Interrogation of Melanoma for Identification of Driver Oncogenic Factors by Rohit Gupta A dissertation submitted in partial fulfillment for the degree of Doctor of Philosophy in the Program for Quantitative & Systems Biology School of Natural Sciences August 2019 Committee in charge: Professor Miriam Barlow, Advisor Professor Michael Colvin, Chair Professor Suzanne Sindi Professor Mark Sistrom Copyright Rohit Gupta, 2019 All rights reserved The Dissertation of Rohit Gupta is approved, and it is acceptable in quality and form for publication on microfilm and electronically: Professor Miriam Barlow, Advisor Professor Michael Colvin, Chair Professor Suzanne Sindi Professor Mark Sistrom University of California, Merced 2019 \To reach a port we must set sail { Sail, not tie at anchor Sail, not drift." Franklin D. Roosevelt UNIVERSITY OF CALIFORNIA, MERCED Abstract Program for Quantitative & Systems Biology School of Natural Sciences Doctor of Philosophy by Rohit Gupta Underpinning genomic principles are defining feature in every physiologic and pathologic process. Through advances in metabolomics, systems biologists can now track the dynamic interactions of the metabolome with the epigenome, genome, transcriptome and proteome. Understanding of cross talk between genomic, epige- nomic and structural changes at biophysical scale on cellular metabolism is still in its infancy. Using Next-Gen Sequencing data in tandem with biophysical approaches, metabolism was found to play an important role in cellular prolif- eration, differentiation, metastasis. -
Deciphering the Long Non-Coding Rnas and Micrornas Coregulation Networks in Ovarian Cancer Development: an Overview
cells Review Deciphering the Long Non-Coding RNAs and MicroRNAs Coregulation Networks in Ovarian Cancer Development: An Overview César López-Camarillo 1,2,* , Erika Ruíz-García 2,3 , Yarely M. Salinas-Vera 4 , Macrina B. Silva-Cázares 5 , Olga N. Hernández-de la Cruz 1, Laurence A. Marchat 6 and Dolores Gallardo-Rincón 2,3 1 Posgrado en Ciencias Genómicas, Universidad Autónoma de la Ciudad de México, 03100 CDMX, Mexico; [email protected] 2 Grupo de Investigación en Cáncer de Ovario y Endometrio, Instituto Nacional de Cancerología, 14080 CDMX, Mexico; [email protected] (E.R.-G.); [email protected] (D.G.-R.) 3 Laboratorio de Medicina Traslacional y Departamento de Tumores Gastrointestinales, Instituto Nacional de Cancerología, 14080 CDMX, Mexico 4 Departamento de Bioquímica, CINVESTAV-IPN, 07360 CDMX, Mexico; [email protected] 5 Coordinación Académica Región Altiplano, Universidad Autónoma de San Luis Potosí, 78700 San Luis Potosí, Mexico; [email protected] 6 Programa en Biomedicina Molecular y Red de Biotecnología, Instituto Politécnico Nacional, 07340 CDMX, Mexico; [email protected] * Correspondence: [email protected] Abstract: Non-coding RNAs are emergent elements from the genome, which do not encode for proteins but have relevant cellular functions impacting almost all the physiological processes oc- Citation: López-Camarillo, C.; curring in eukaryotic cells. In particular, microRNAs and long non-coding RNAs (lncRNAs) are a Ruíz-García, E.; Salinas-Vera, Y.M.; new class of small RNAs transcribed from the genome, which modulate the expression of specific Silva-Cázares, M.B.; Hernández-de la Cruz, O.N.; Marchat, L.A.; genes at transcriptional and posttranscriptional levels, thus adding a new regulatory layer in the flux Gallardo-Rincón, D. -
Comparative Proteomic and Regulatory Network Analyses of the Elongating Pig Conceptus
2678 DOI 10.1002/pmic.200800776 Proteomics 2009, 9, 2678–2694 RESEARCH ARTICLE Comparative proteomic and regulatory network analyses of the elongating pig conceptus Séverine A. Degrelle1*, Le Ann Blomberg1, Wesley M. Garrett1, Robert W. Li2 and Neil C. Talbot1 1 USDA Agricultural Research Service, Animal and Natural Resources Institute, Animal Biosciences and Biotechnology Laboratory, Beltsville, MD, USA 2 Bovine Functional Genomics Laboratory, Beltsville, MD, USA Embryo loss during peri-implantation can approach 20% in swine following artificial insemina- Received: September 30, 2008 tion or natural mating and coincides with rapid conceptus elongation. The objective of the pres- Revised: December 31, 2008 ent study was to establish a comprehensive profile of the abundant proteins of the pig conceptus Accepted: January 13, 2009 at the time prior to implantation and identify stage-specific changes during elongation. The abundant proteins of a homogenous population of gestational day-11 ovoid (0.7–1 cm) and gestational day-12 filamentous (15–20 cm) porcine concepti were compared by extracting pro- teins from three independent conceptus pools and separating the proteins by 2-DE. Proteins in 305 spots were analyzed by MALDI-TOF or additionally by LC-MS/MS and 275 were positively identified representing 174 distinct proteins. The proteins could be classified into the following functional categories: cell proliferation/differentiation, cytoskeleton, metabolism, and stress re- sponse. Based on spot density, 35 proteins associated with cell proliferation, differentiation, apoptosis, and embryo/maternal signaling, were found to be differentially expressed between ovoid and filamentous concepti. A comparison of the protein expression profile with tran- scriptomic data from pig concepti of the same developmental stages identified similarities and dissimilarities between protein and mRNA expression profiles. -
Genome-Scale Models of Metabolism and Gene Expression : : Construction and Use for Growth Phenotype Prediction
UC San Diego UC San Diego Electronic Theses and Dissertations Title Genome-scale Models of Metabolism and Gene Expression : : Construction and Use for Growth Phenotype Prediction Permalink https://escholarship.org/uc/item/8zq4p227 Author Lerman, Joshua Adam Publication Date 2014 Peer reviewed|Thesis/dissertation eScholarship.org Powered by the California Digital Library University of California UNIVERSITY OF CALIFORNIA, SAN DIEGO Genome-scale Models of Metabolism and Gene Expression: Construction and Use for Growth Phenotype Prediction A dissertation submitted in partial satisfaction of the requirements for the degree Doctor of Philosophy in Bioinformatics & Systems Biology by Joshua Adam Lerman Committee in charge: Professor Bernhard Ø. Palsson, Chair Professor Milton H. Saier, Jr., Co-Chair Professor Philip E. Bourne Professor Terence Hwa Professor Victor Nizet 2014 Copyright Joshua Adam Lerman, 2014 All rights reserved. The dissertation of Joshua Adam Lerman is approved, and it is acceptable in quality and form for publication on microfilm and electronically: Co-Chair Chair University of California, San Diego 2014 iii DEDICATION To my mother and father, for your love, guidance, and all the sacrifices you made for Justin, Rachel, and I. To Lauren, for your love and all those times I told you, \One sec." To the loving memory of Bubby. iv EPIGRAPH Tony Stark was able to build this in a cave! WITH A BOX OF SCRAPS!! |Obadiah Stane, Iron Man v TABLE OF CONTENTS Signature Page................................. iii Dedication................................... -
Astrocytes Close the Critical Period for Visual Plasticity
bioRxiv preprint doi: https://doi.org/10.1101/2020.09.30.321497; this version posted October 2, 2020. The copyright holder for this preprint (which was not certified by peer review) is the author/funder. All rights reserved. No reuse allowed without permission. 1 Astrocytes close the critical period for visual plasticity 2 3 Jérôme Ribot1‡, Rachel Breton1,2,3‡#, Charles-Félix Calvo1, Julien Moulard1,4, Pascal Ezan1, 4 Jonathan Zapata1, Kevin Samama1, Alexis-Pierre Bemelmans5, Valentin Sabatet6, Florent 5 Dingli6, Damarys Loew6, Chantal Milleret1, Pierre Billuart7, Glenn Dallérac1£#, Nathalie 6 Rouach1£* 7 8 1Neuroglial Interactions in Cerebral Physiology, Center for Interdisciplinary Research in 9 Biology, Collège de France, CNRS UMR 7241, INSERM U1050, Labex Memolife, PSL 10 Research University Paris, France 11 12 2Doctoral School N°568, Paris Saclay University, PSL Research University, Le Kremlin 13 Bicetre, France 14 15 3Astrocytes & Cognition, Paris-Saclay Institute for Neurosciences, CNRS UMR 9197, Paris- 16 Saclay University, Orsay, France 17 18 4Doctoral School N°158, Sorbonne University, Paris France 19 20 5Commissariat à l’Energie Atomique et aux Energies Alternatives (CEA), Département de la 21 Recherche Fondamentale, Institut de biologie François Jacob, MIRCen, and CNRS UMR 22 9199, Université Paris-Saclay, Neurodegenerative Diseases Laboratory, Fontenay-aux-Roses, 23 France 24 25 6Institut Curie, PSL Research University, Mass Spectrometry and Proteomics Laboratory, 26 Paris, France 27 28 7Université de Paris, Institute of -
Genetic Testing for Non-Cancerous Inheritable Diseases
Genetic Testing for Non-Cancerous Inheritable Diseases Policy Number: Original Effective Date: MM.02.009 03/01/2010 Line(s) of Business: Current Effective Date: HMO; PPO; QUEST 11/01/2013 Section: Medicine Place(s) of Service: Outpatient I. Description A genetic test is the analysis of human DNA, RNA, chromosomes, proteins, or certain metabolites in order to detect alterations related to an inheritable disorder. This can be accomplished by directly examining the DNA or RNA that makes up a gene (direct testing), looking at markers co-inherited with a disease-causing gene (linkage testing), assaying certain metabolites (biochemical testing), or examining the chromosomes (cytogenetic testing). Genetic tests are conducted for a number of purposes, including predicting disease risk, newborn screening, determining clinical management, identifying carriers, and establishing prenatal or clinical diagnoses or prognoses in individuals, families, or populations. For the purpose of this policy, first-degree relatives are defined as parents, full siblings, and offspring. Second-degree relatives are defined as grandparents, grandchildren, aunts, uncles, nephews, nieces, half-siblings and third-degree relatives are defined as great-grandparents, great-aunts, great-uncles, first cousins. This policy does not address oncology-related genetic testing or pre-implantation genetic diagnosis (PGD). II. Criteria/Guidelines A. Genetic testing for all the inheritable diseases listed below must meet the following factors (in addition to any specific criteria) in order to be covered: 1. There must be a reasonable expectation based on family history, pedigree analysis, risk factors, and/or symptomatology that a genetically inherited condition exists. 2. The genotypes to be detected by a genetic test must be shown by scientifically valid methods to be associated with the occurrence of the disease. -
Method for a Genome Wide Identification of Expression
(19) TZZ ___T (11) EP 2 177 615 A1 (12) EUROPEAN PATENT APPLICATION (43) Date of publication: (51) Int Cl.: 21.04.2010 Bulletin 2010/16 C12N 15/11 (2006.01) (21) Application number: 08075816.2 (22) Date of filing: 10.10.2008 (84) Designated Contracting States: • Weltmeier, Fridtjof, Dr. AT BE BG CH CY CZ DE DK EE ES FI FR GB GR 30627 Hannover (DE) HR HU IE IS IT LI LT LU LV MC MT NL NO PL PT RO SE SI SK TR (74) Representative: Baumbach, Friedrich Designated Extension States: Patentanwalt AL BA MK RS Robert-Rössle-Strasse 10 13125 Berlin (DE) (71) Applicant: Fraunhofer-Gesellschaft zur Förderung der Remarks: angewandten Forschung e.V. Claims 16-27,29,31,33-44 and 46-47 are deemed to 80686 München (DE) be abandoned due to non-payment of the claims fees (Rule 45(3) EPC). (72) Inventors: • Borlak, Jürgen, Prof. Dr. 31275 Lehrte OT Immensen (DE) (54) Method for a genome wide identification of expression regulatory sequences and use of genes and molecules derived thereof for the diagnosis and therapy of metabolic and/or tumorous diseases (57) The invention is directed to the use of particular located within the chromosomal position specified by par- human genes, nucleic acids hybridizing to said genes, ticular start and end sites. and gene products encoded thereby in the context of the The invention further relates to a method for a diagnosis and/or therapy of metabolic and/or cancerous genomewide identification of functional binding sites at diseases, preferably of diabetes mellitus and/or colorec- specifically targeted DNA sequences with high resolu- tal cancer, wherein the gene is selected from the group tion, wherein the method comprises, or preferably con- of the human chromosomal genes having at least one sists of, the steps of: expression regulatory sequence according to matrix 1 a. -
Download Validation Data
PrimePCR™Assay Validation Report Gene Information Gene Name cytidine monophosphate (UMP-CMP) kinase 1, cytosolic Gene Symbol CMPK1 Organism Human Gene Summary This gene encodes one of the enzymes required for cellular nucleic acid biosynthesis. This enzyme catalyzes the transfer of a phosphate group from ATP to CMP UMP or dCMP to form the corresponding diphosphate nucleotide. Alternate splicing results in both coding and non-coding transcript variants. Gene Aliases CMK, CMPK, UMK, UMP-CMPK, UMPK RefSeq Accession No. NC_000001.10, NT_032977.9 UniGene ID Hs.731647 Ensembl Gene ID ENSG00000162368 Entrez Gene ID 51727 Assay Information Unique Assay ID qHsaCED0038110 Assay Type SYBR® Green Detected Coding Transcript(s) ENST00000546544, ENST00000538496, ENST00000341771, ENST00000372086, ENST00000372084, ENST00000292609, ENST00000371873, ENST00000471289, ENST00000450808, ENST00000360737, ENST00000393235, ENST00000464064, ENST00000358127, ENST00000332312, ENST00000409816, ENST00000409090, ENST00000389524, ENST00000428314, ENST00000407327, ENST00000335757, ENST00000586078, ENST00000330394, ENST00000355502, ENST00000396879, ENST00000329271 Amplicon Context Sequence TCCTGGGCCTTAGCTTCCTGCTGCAGACCCGCCGGCCGATTCTCCTCTGCTCTC CACGTCTCATGAAGCCGCTGGTCGTGTTCGTCCTCGGCGGC Amplicon Length (bp) 65 Chromosome Location 1:47799652-47799746 Assay Design Exonic Purification Desalted Validation Results Efficiency (%) 101 R2 0.9995 cDNA Cq 19.96 Page 1/5 PrimePCR™Assay Validation Report cDNA Tm (Celsius) 84.5 gDNA Cq 29.09 Specificity (%) 100 Information to assist with -
Genomic Approaches Uncover Increasing Complexities in the Regulatory Landscape at the Human SCL (TAL1) Locus
Genomic Approaches Uncover Increasing Complexities in the Regulatory Landscape at the Human SCL (TAL1) Locus Pawandeep Dhami1¤a, Alexander W. Bruce1¤b, Johanna H. Jim1¤c, Shane C. Dillon1¤d, Amanda Hall1, Jonathan L. Cooper1, Nicolas Bonhoure2, Kelly Chiang2, Peter D. Ellis1, Cordelia Langford1, Robert M. Andrews1, David Vetrie1,2* 1 The Wellcome Trust Sanger Institute, Hinxton, United Kingdom, 2 Section of Pathology and Gene Regulation, Division of Cancer Sciences and Molecular Pathology, University of Glasgow, Glasgow, United Kingdom Abstract The SCL (TAL1) transcription factor is a critical regulator of haematopoiesis and its expression is tightly controlled by multiple cis-acting regulatory elements. To elaborate further the DNA elements which control its regulation, we used genomic tiling microarrays covering 256 kb of the human SCL locus to perform a concerted analysis of chromatin structure and binding of regulatory proteins in human haematopoietic cell lines. This approach allowed us to characterise further or redefine known human SCL regulatory elements and led to the identification of six novel elements with putative regulatory function both up and downstream of the SCL gene. They bind a number of haematopoietic transcription factors (GATA1, E2A LMO2, SCL, LDB1), CTCF or components of the transcriptional machinery and are associated with relevant histone modifications, accessible chromatin and low nucleosomal density. Functional characterisation shows that these novel elements are able to enhance or repress SCL promoter activity, have endogenous promoter function or enhancer-blocking insulator function. Our analysis opens up several areas for further investigation and adds new layers of complexity to our understanding of the regulation of SCL expression. -
Signalling and Genome-Wide Transcriptional Regulation in Fast Muscle of Zebrafish Following a Single-Satiating Meal
2125 The Journal of Experimental Biology 214, 2125-2139 © 2011. Published by The Company of Biologists Ltd doi:10.1242/jeb.053298 RESEARCH ARTICLE Insulin-like growth factor (IGF) signalling and genome-wide transcriptional regulation in fast muscle of zebrafish following a single-satiating meal Ian P. G. Amaral* and Ian A. Johnston Scottish Oceans Institute, School of Biology, University of St Andrews, St Andrews, Fife KY16 8LB, UK *Author for correspondence ([email protected]) Accepted 8 March 2011 SUMMARY Male zebrafish (Danio rerio) were fasted for 7days and fed to satiation over 3h to investigate the transcriptional responses to a single meal. The intestinal content at satiety (6.3% body mass) decreased by 50% at 3h and 95% at 9h following food withdrawal. Phosphorylation of the insulin-like growth factor (IGF) signalling protein Akt peaked within 3h of feeding and was highly correlated with gut fullness. Retained paralogues of IGF hormones genes were regulated with feeding, with igf1a showing a pronounced peak in expression after 3h and igf2b after 6h. Igf-I receptor transcripts were markedly elevated with fasting, and decreased to their lowest levels 45min after feeding. igf1rb transcripts increased more quickly than igf1ra transcripts as the gut emptied. Paralogues of the insulin-like growth factor binding proteins (IGFBPs) were constitutively expressed, except for igfbp1a and igfbp1b transcripts, which were significantly elevated with fasting. Genome-wide transcriptional responses were analysed using the Agilent 44K oligonucleotide microarray and selected genes validated by qPCR. Fasting was associated with the upregulation of genes for the ubiquitin-proteasome degradation pathway, anti-proliferative and pro-apoptotic genes.