The Human Posterior Superior Temporal Sulcus Samples Visual Space Differently from Other Face-Selective Regions
Total Page:16
File Type:pdf, Size:1020Kb
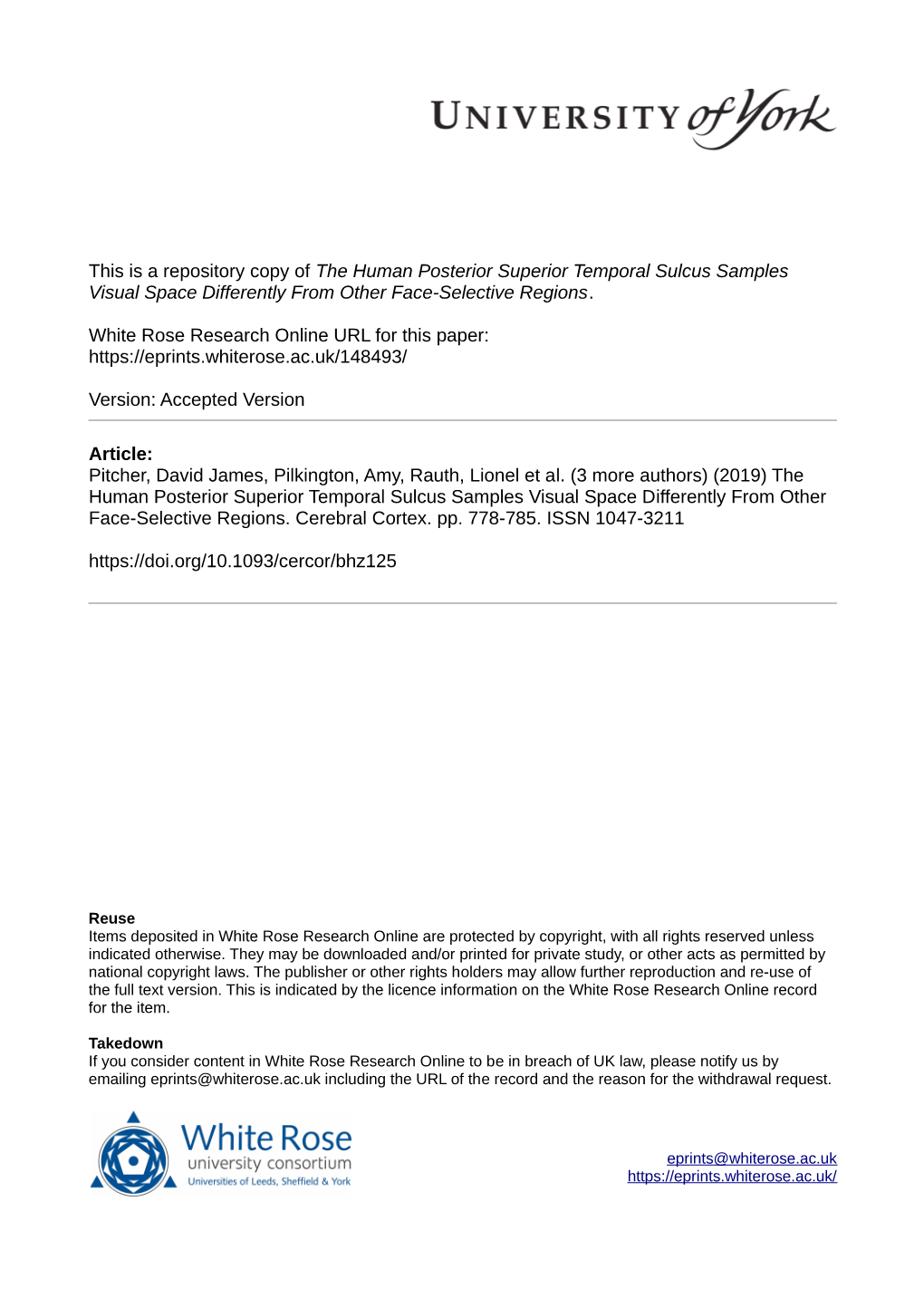
Load more
Recommended publications
-
And Scene-Selective Brain Areas Based on Their
www.nature.com/scientificreports OPEN A functional dissociation of face-, body- and scene-selective brain areas based on their response to Received: 2 March 2018 Accepted: 7 May 2019 moving and static stimuli Published: xx xx xxxx David Pitcher1, Geena Ianni2,3 & Leslie G. Ungerleider3 The human brain contains areas that respond selectively to faces, bodies and scenes. Neuroimaging studies have shown that a subset of these areas preferentially respond more to moving than static stimuli, but the reasons for this functional dissociation remain unclear. In the present study, we simultaneously mapped the responses to motion in face-, body- and scene-selective areas in the right hemisphere using moving and static stimuli. Participants (N = 22) were scanned using functional magnetic resonance imaging (fMRI) while viewing videos containing bodies, faces, objects, scenes or scrambled objects, and static pictures from the beginning, middle and end of each video. Results demonstrated that lateral areas, including face-selective areas in the posterior and anterior superior temporal sulcus (STS), the extrastriate body area (EBA) and the occipital place area (OPA) responded more to moving than static stimuli. By contrast, there was no diference between the response to moving and static stimuli in ventral and medial category-selective areas, including the fusiform face area (FFA), occipital face area (OFA), amygdala, fusiform body area (FBA), retrosplenial complex (RSC) and parahippocampal place area (PPA). This functional dissociation between lateral and ventral/medial brain areas that respond selectively to diferent visual categories suggests that face-, body- and scene- selective networks may be functionally organized along a common dimension. Neuroimaging studies report multiple areas in the human brain that selectively respond to faces, bodies and scenes. -
The Functional Neuroanatomy of Human Face Perception
VS03CH08-GrillSpector ARI 11 August 2017 6:36 Annual Review of Vision Science The Functional Neuroanatomy of Human Face Perception Kalanit Grill-Spector,1,2 Kevin S. Weiner,1 Kendrick Kay,3 and Jesse Gomez4 1Department of Psychology, Stanford University, Stanford, California 94305; email: [email protected] 2Stanford Neurosciences Institute, Stanford University, Stanford, California 94305 3Department of Radiology, University of Minnesota, Minneapolis, Minnesota 55455 4Neurosciences Program, Stanford University School of Medicine, Stanford, California 94305 Annu. Rev. Vis. Sci. 2017. 3:167–96 Keywords First published as a Review in Advance on July 17, face recognition, fMRI, face network, FFA, ventral stream, mid-fusiform 2017 sulcus, population receptive fields The Annual Review of Vision Science is online at vision.annualreviews.org Abstract Access provided by 76.21.3.180 on 09/20/17. For personal use only. https://doi.org/10.1146/annurev-vision-102016- Face perception is critical for normal social functioning and is mediated Annu. Rev. Vis. Sci. 2017.3:167-196. Downloaded from www.annualreviews.org 061214 by a network of regions in the ventral visual stream. In this review, we Copyright c 2017 by Annual Reviews. ⃝ describe recent neuroimaging findings regarding the macro- and micro- All rights reserved scopic anatomical features of the ventral face network, the characteristics of white matter connections, and basic computations performed by population ANNUAL receptive fields within face-selective regions composing this network. We REVIEWS Further emphasize the importance of the neural tissue properties and white matter Click here to view this article's online features: connections of each region, as these anatomical properties may be tightly t%PXOMPBEmHVSFTBT115TMJEFT linked to the functional characteristics of the ventral face network. -
A Network Linking Scene Perception and Spatial Memory Systems in Posterior Cerebral Cortex ✉ Adam Steel 1 , Madeleine M
ARTICLE https://doi.org/10.1038/s41467-021-22848-z OPEN A network linking scene perception and spatial memory systems in posterior cerebral cortex ✉ Adam Steel 1 , Madeleine M. Billings1, Edward H. Silson2 & Caroline E. Robertson 1 The neural systems supporting scene-perception and spatial-memory systems of the human brain are well-described. But how do these neural systems interact? Here, using fine-grained individual-subject fMRI, we report three cortical areas of the human brain, each lying 1234567890():,; immediately anterior to a region of the scene perception network in posterior cerebral cortex, that selectively activate when recalling familiar real-world locations. Despite their close proximity to the scene-perception areas, network analyses show that these regions constitute a distinct functional network that interfaces with spatial memory systems during naturalistic scene understanding. These “place-memory areas” offer a new framework for understanding how the brain implements memory-guided visual behaviors, including navigation. 1 Department of Psychology and Brain Sciences, Dartmouth College, Hanover, NH, USA. 2 Psychology, School of Philosophy, Psychology, and Language ✉ Sciences, University of Edinburgh, Edinburgh EH8 9JZ, UK. email: [email protected] NATURE COMMUNICATIONS | (2021) 12:2632 | https://doi.org/10.1038/s41467-021-22848-z | www.nature.com/naturecommunications 1 ARTICLE NATURE COMMUNICATIONS | https://doi.org/10.1038/s41467-021-22848-z s we navigate through our world, the visual scene in front network properties of these “place-memory areas” (Experiments 2–3) Aof us is seamlessly integrated with our memory of the and explicitly tested the wide-spread view that the neural substrates broader spatial environment. -
Mechanisms of Hemispheric Lateralization: Asymmetric Interhemispheric Recruitment in the Face Perception Network
NeuroImage 124 (2016) 977–988 Contents lists available at ScienceDirect NeuroImage journal homepage: www.elsevier.com/locate/ynimg Mechanisms of hemispheric lateralization: Asymmetric interhemispheric recruitment in the face perception network Stefan Frässle a,b,⁎, Frieder Michel Paulus c, Sören Krach c, Stefan Robert Schweinberger d, Klaas Enno Stephan e,f, Andreas Jansen a a Laboratory for Multimodal Neuroimaging (LMN), Department of Psychiatry, University of Marburg, D-35039 Marburg, Germany b Department of Child- and Adolescent Psychiatry, University of Marburg, D-35039 Marburg, Germany c Department of Psychiatry and Psychotherapy, Social Neuroscience Lab|SNL, University of Lübeck, D-23538 Lübeck, Germany d Department of General Psychology and Cognitive Neuroscience, Institute of Psychology, University of Jena, D-07743 Jena, Germany e Translational Neuromodeling Unit (TNU), Institute of Biomedical Engineering, University of Zurich & ETH Zurich, CH-8032 Zurich, Switzerland f Wellcome Trust Centre for Neuroimaging, University College London, London WC1N 3BG, United Kingdom article info abstract Article history: Perceiving human faces constitutes a fundamental ability of the human mind, integrating a wealth of information Received 3 July 2015 essential for social interactions in everyday life. Neuroimaging studies have unveiled a distributed neural net- Accepted 26 September 2015 work consisting of multiple brain regions in both hemispheres. Whereas the individual regions in the face per- Available online 9 October 2015 ception network and the right-hemispheric dominance for face processing have been subject to intensive research, the functional integration among these regions and hemispheres has received considerably less atten- Keywords: Dynamic causal modeling tion. Using dynamic causal modeling (DCM) for fMRI, we analyzed the effective connectivity between the core Face perception regions in the face perception network of healthy humans to unveil the mechanisms underlying both intra- Fusiform face area and interhemispheric integration. -
Functional Disconnection Between the Visual Cortex and Right Fusiform Face Area in Schizophrenia
Schizophrenia Research 209 (2019) 72–79 Contents lists available at ScienceDirect Schizophrenia Research journal homepage: www.elsevier.com/locate/schres Functional disconnection between the visual cortex and right fusiform face area in schizophrenia S. Maher ⁎, T. Ekstrom, D. Ongur, D.L. Levy, D.J. Norton, L.D. Nickerson 1, Y. Chen 1,2 McLean Hospital, Harvard Medical School, United States of America article info abstract Article history: Patients with schizophrenia show impairment in processing faces, including facial affect and face detection, but Received 31 October 2018 the underlying mechanisms are unknown. We used functional magnetic resonance imaging (fMRI) to character- Received in revised form 28 March 2019 ize resting state functional connectivity between an independent component analysis (ICA)-defined early visual Accepted 6 May 2019 cortical network (corresponding to regions in V1, V2, V3) and aprioridefined face-processing regions (fusiform Available online 22 May 2019 face area [FFA], occipital face area [OFA], superior temporal sulcus [STS] and amygdala) using dual regression in Keywords: 20 schizophrenia patients and 26 healthy controls. We also investigated the association between resting func- Face processing tional connectivity and neural responses (fMRI) elicited by a face detection paradigm in a partially overlapping Brain connectivity sample (Maher et al., 2016) that used stimuli equated for lower-level perceptual abilities. Group differences in Resting state functional connectivity were found in right FFA only; controls showed significantly stronger functional connec- Schizophrenia tivity to an early visual cortical network. Functional connectivity in right FFA was associated with (a) neural re- sponses during face detection in controls only, and (b) perceptual detection thresholds for faces in patients only. -
Schaer K., Jahn G., Lotze M. (2012) Fmri-Activation During Drawing A
Behavioural Brain Research 233 (2012) 209–216 Contents lists available at SciVerse ScienceDirect Behavioural Brain Research j ournal homepage: www.elsevier.com/locate/bbr Research report fMRI-activation during drawing a naturalistic or sketchy portrait a b a,∗ K. Schaer ,G.Jahn , M. Lotze a Functional Imaging Unit, Center for Diagnostic Radiology and Neuroradiology, University of Greifswald, Greifswald, Germany b Department of Psychology, University of Greifswald, Greifswald, Germany h i g h l i g h t s We used fMRI to measure 20 naive subjects during drawing a portrait. Participants were able to track their drawing online. We identified three important circuits specific for the process of portrait drawing. Circuits where: face perception, location encoding, and continuous feedback processes. Representations involved: fusiform gyrus, precuneus, parietal sulcus, and cerebellum. a r t i c l e i n f o a b s t r a c t Article history: Neural processes for naturalistic drawing might be discerned into object recognition and analysis, atten- Received 8 March 2012 tion processes guiding eye hand interaction, encoding of visual features in an allocentric reference frame, Received in revised form 3 May 2012 a transfer into the motor command and precise motor guidance with tight sensorimotor feedback. Cere- Accepted 8 May 2012 bral representations in a real life paradigm during naturalistic drawing have sparsely been investigated. Available online 15 May 2012 Using a functional Magnetic Resonance Imaging (fMRI) paradigm we measured 20 naive subjects during drawing a portrait from a frontal face presented as a photograph. Participants were asked to draw the Keywords: portrait in either a naturalistic or a sketchy characteristic way. -
The Functional Neuroanatomy of Face Processing: Insights from Neuroimaging and Implications for Deep Learning
Chapter 1 The Functional Neuroanatomy of Face Processing: Insights from Neuroimaging and Implications for Deep Learning Kalanit Grill-Spector, Kendrick Kay and Kevin S. Weiner Abstract Face perception is critical for normal social functioning, and is mediated by a cortical network of regions in the ventral visual stream. Comparative analysis between present deep neural network architectures for biometrics and neural archi- tectures in the human brain is necessary for developing artificial systems with human abilities. Neuroimaging research has advanced our understanding regarding the func- tional architecture of the human ventral face network. Here, we describe recent neu- roimaging findings in three domains: (1) the macro- and microscopic anatomical features of the ventral face network in the human brain, (2) the characteristics of white matter connections, and (3) the basic computations performed by population receptive fields within face-selective regions composing this network. Then, we con- sider how empirical findings can inform the development of accurate computational deep neural networks for face recognition as well as shed light on computational benefits of specific neural implementational features. Introduction Face perception is critical for normal social functioning. For example, faces pro- vide key visual information that we use to discriminate one person from another every single day. Since face perception is ecologically and evolutionarily relevant across species [39, 52, 152, 167] a fundamental question is: What neuroanatomical and functional features of the human brain contribute to the visual perception and recognition of faces? K. Grill-Spector (B) · K.S. Weiner Department of Psychology, Stanford University, Stanford, CA, USA e-mail: [email protected] K. -
Prosopagnosia By: Leah Fleischman Leah Graduated in September 2014 with B.S
Prosopagnosia By: Leah Fleischman Leah graduated in September 2014 with B.S. in biology. Abstract Prosopagnosia is a cognitive disorder that affects one’s ability to recognize faces. Prosopagnosia can be caused by a congenital defect, or it can be acquired as a result of brain damage. Much research has been devoted to discovering the specific causes and effects of Prosopagnosia. Many case studies have been performed in order to determine the specific effects that each case of Prosopagnosia causes for various individuals suffering from the disease. This article discusses the various aspects of Prosopagnosia; specifically focusing on the behavioral, anatom- ical, and neurological implications. Introduction Prosopagnosia, also called face-blindness, is a cognitive disorder of are usually able to acknowledge that they are looking at a face; face perception. A person’s ability to recognize faces is impaired, however they are unable to identify the specific face. As a result, while other aspects of visual processing and intellectual func- they have come to rely on other cues such as voices, clothing, tioning may remain intact. There are two types of Prosopagnosia: or specific accessories (hairlines, eyebrow shapes, etc.) to help Congenital or Developmental Prosopagnosia, and Acquired recognize a person. CP can even affect the recognition of even Prosopagnosia. Congenital Prosopagnosia is a face-recognition the most familiar faces, such as close family members. In extreme deficit that is life long, beginning in early childhood, and is not cases, one may not even be able to recognize his- or herself. An attributed to brain damage. Acquired Prosopagnosia refers to a important fact to understand regarding those with CP is that condition that follows acute brain damage, usually brain damage they have never experienced normal face perception. -
Social Perception of Faces: Brain Imaging and Subjective Ratings
brain sciences Article Social Perception of Faces: Brain Imaging and Subjective Ratings Peter Walla 1,2,3,4,* , Minah Chang 1, Katrin Schaefer 5 and Sonja Windhager 5 1 CanBeLab, Department of Psychology, Webster Vienna Private University, Palais Wenkheim, Praterstrasse 23, 1020 Vienna, Austria; [email protected] 2 School of Psychology, Centre for Translational Neuroscience and Mental Health Research, University of Newcastle, University Drive, Callaghan 2308, NSW, Australia 3 Faculty of Psychology, Sigmund Freud University, Freudplatz 1, 1020 Vienna, Austria 4 Faculty of Medicine, Sigmund Freud University, Freudplatz 3, 1020 Vienna, Austria 5 Department of Evolutionary Anthropology, University of Vienna, Althanstrasse 14, 1090 Vienna, Austria; [email protected] (K.S.); [email protected] (S.W.) * Correspondence: [email protected]; Tel.: +43-1-7984098 Received: 28 March 2020; Accepted: 5 November 2020; Published: 16 November 2020 Abstract: The aim of this study was to investigate how a female face is perceived in terms of its attractiveness, dominance, health, femininity-masculinity, and maturity in direct relation to the body fat percentage (BFP) conveyed by the face. To compare how young adults (ages 18 to 35) respond to different levels of body fat percentage both subjectively and objectively we collected survey ratings and electroencephalography (EEG) data across five different levels of BFP from 40 participants. We adapted the experimental design from a prior behavioral study and used calibrated and morphed female face images of five different BFP levels. The results of the survey are in consensus with the previous study and assessed to be a successful replication. -
The Functional Neuroanatomy of Human Face Perception
VS03CH08-GrillSpector ARI 11 August 2017 6:36 Annual Review of Vision Science The Functional Neuroanatomy of Human Face Perception Kalanit Grill-Spector,1,2 Kevin S. Weiner,1 Kendrick Kay,3 and Jesse Gomez4 1Department of Psychology, Stanford University, Stanford, California 94305; email: [email protected] 2Stanford Neurosciences Institute, Stanford University, Stanford, California 94305 3Department of Radiology, University of Minnesota, Minneapolis, Minnesota 55455 4Neurosciences Program, Stanford University School of Medicine, Stanford, California 94305 Annu. Rev. Vis. Sci. 2017. 3:167–96 Keywords First published as a Review in Advance on July 17, face recognition, fMRI, face network, FFA, ventral stream, mid-fusiform 2017 sulcus, population receptive fields The Annual Review of Vision Science is online at vision.annualreviews.org Abstract https://doi.org/10.1146/annurev-vision-102016- Face perception is critical for normal social functioning and is mediated Annu. Rev. Vis. Sci. 2017.3:167-196. Downloaded from www.annualreviews.org 061214 by a network of regions in the ventral visual stream. In this review, we Copyright c 2017 by Annual Reviews. describe recent neuroimaging findings regarding the macro- and micro- All rights reserved scopic anatomical features of the ventral face network, the characteristics of white matter connections, and basic computations performed by population Access provided by University of Minnesota - Twin Cities Law Library on 10/03/17. For personal use only. ANNUAL receptive fields within face-selective regions composing this network. We REVIEWS Further emphasize the importance of the neural tissue properties and white matter Click here to view this article's online features: connections of each region, as these anatomical properties may be tightly • Download figures as PPT slides linked to the functional characteristics of the ventral face network. -
Isolated Prosopagnosia As the Presenting Complaint in Glioblastoma the Face of Deception
RESIDENT & FELLOW SECTION Pearls & Oy-sters: Isolated prosopagnosia as the presenting complaint in glioblastoma The face of deception Saurav Das, MD, and Eric Burton, MD Correspondence Dr. Das Neurology 2019;93:642-644. doi:10.1212/WNL.0000000000008204 ® [email protected] Pearls c Prosopagnosia localizes to the fusiform gyrus in the medial temporal lobe and occurs from bilateral lesions or less frequently from unilateral lesions involving the right side alone. c Commonly reported lesions resulting in prosopagnosia include strokes and seizures involving the above regions, while tumors are a rarely reported cause. c Facial recognition is a more complex process than previously thought. Core face recognition network in the brain includes the occipital face area, the fusiform face area, and the face-selective posterior superior temporal sulcus. Oy-sters c An isolated cognitive deficit like prosopagnosia may be the first presenting symptom in patients with primary brain tumors or metastatic disease that involves the bilateral medial temporal lobes or the right medial temporal lobe alone. c Detailed neurologic and ophthalmologic examinations can have localization value, as in this case of rare neuro-behavioral syndrome associated with a focal brain lesion. Prosopagnosia is the inability to recognize familiar faces. Patients with this disorder usually retain otherwise normal visual function and are generally able to recognize other objects. Typically patients are distressed because they cannot recognize family, friends, and public figures by face. Lesions in the medial temporal lobe, specifically the temporo-occipital area (fusiform gyrus), are sufficient to cause the deficit. Interestingly, both bilateral and unilateral right-sided lesions have been associated with prosopagnosia. -
1 Dissociable Pathways for Moving and Static Face Perception Begin In
Dissociable pathways for moving and static face perception begin in early visual cortex: evidence from an acquired prosopagnosic Magdalena W Sliwinska, Caitlin Bearpark, Julia Corkhill, Aimee McPhillips & David Pitcher Department of Psychology, University of York, Heslington, York, YO105DD, U.K. Corresponding author: David Pitcher: [email protected] Department of Psychology, University of York, Heslington, York, YO105DD, U.K Keywords Face processing, occipital face area (OFA), fusiform face area (FFA), posterior superior temporal sulcus (pSTS) Highlights • An acquired prosopagnosic with a right occipitotemporal lesion completed three fMRI experiments • Results showed an impaired response in his right OFA and right FFA • The response in his right pSTS was comparable with controls despite his prosopagnosia. • These results suggest pSTS has functional inputs independent of the OFA. 1 Abstract To investigate the functional connections between the core components of the face processing network we tested Herschel, an acquired prosopagnosic patient with a right ventral occipitotemporal lesion. In Experiment 1 Herschel, and control participants, were scanned with functional magnetic resonance imaging (fMRI) while viewing videos of moving faces or static images taken from the videos. In Experiment 2 participants viewed videos of actors making facial expressions or static images taken from the videos. In Experiment 3, participants viewed videos of moving faces presented in the left or right visual field. Results showed the neural response in Herschel’s right occipital face area (OFA) was impaired for moving and static faces (Experiment 1), moving expressions (Experiment 2) and moving faces in the left visual field (Experiment 3). The response in Herschel’s right fusiform face area (FFA) to moving and static faces was impaired in Experiment 1 only, in Experiments 2 and 3 Herschel’s FFA response was not significantly different from controls.