Greenland Ice Sheet Surface Air Temperature Variability: 1840–2007*
Total Page:16
File Type:pdf, Size:1020Kb
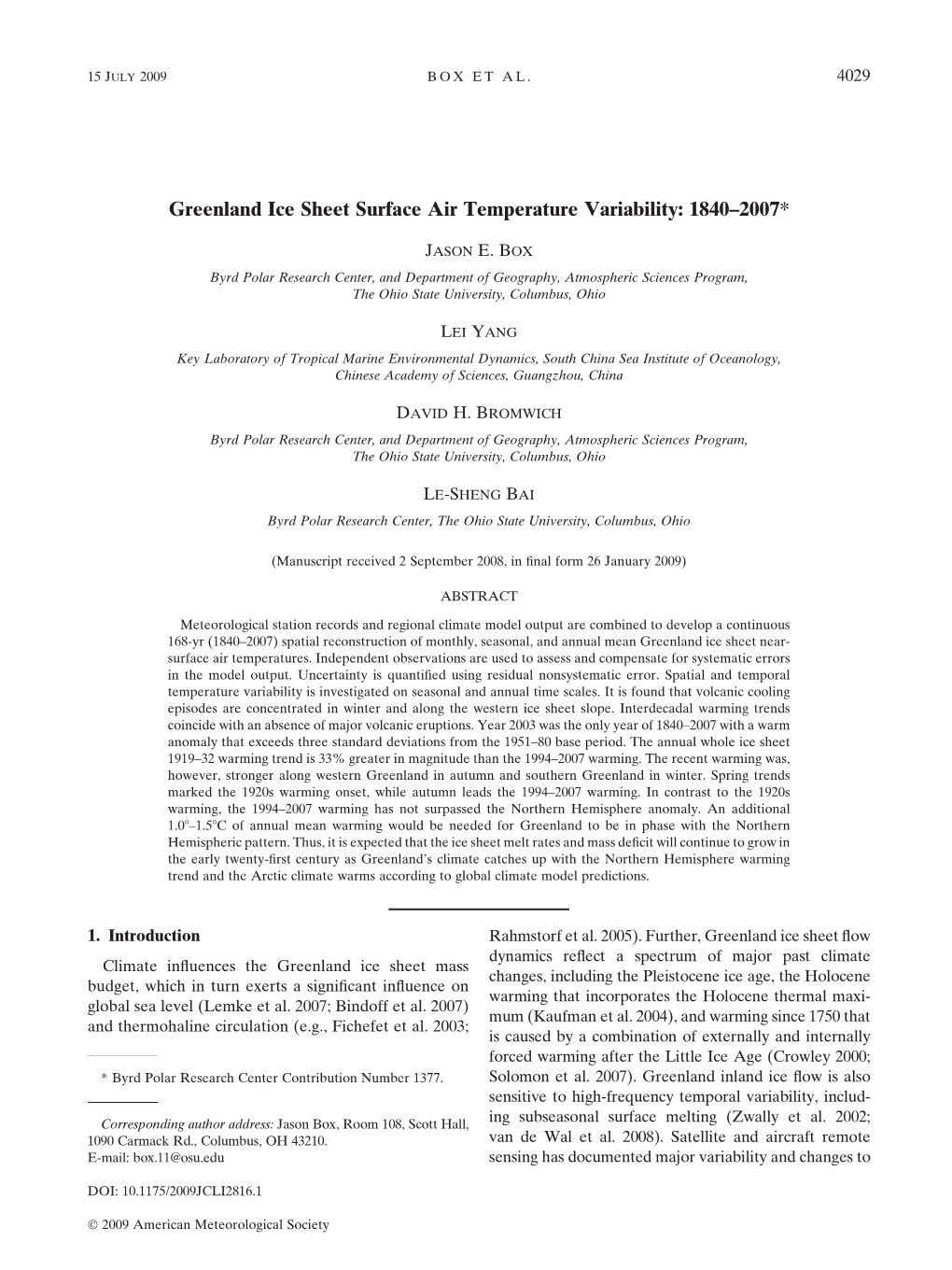
Load more
Recommended publications
-
Natural Resources in the Nanortalik District
National Environmental Research Institute Ministry of the Environment Natural resources in the Nanortalik district An interview study on fishing, hunting and tourism in the area around the Nalunaq gold project NERI Technical Report No. 384 National Environmental Research Institute Ministry of the Environment Natural resources in the Nanortalik district An interview study on fishing, hunting and tourism in the area around the Nalunaq gold project NERI Technical Report No. 384 2001 Christain M. Glahder Department of Arctic Environment Data sheet Title: Natural resources in the Nanortalik district Subtitle: An interview study on fishing, hunting and tourism in the area around the Nalunaq gold project. Arktisk Miljø – Arctic Environment. Author: Christian M. Glahder Department: Department of Arctic Environment Serial title and no.: NERI Technical Report No. 384 Publisher: Ministry of Environment National Environmental Research Institute URL: http://www.dmu.dk Date of publication: December 2001 Referee: Peter Aastrup Greenlandic summary: Hans Kristian Olsen Photos & Figures: Christian M. Glahder Please cite as: Glahder, C. M. 2001. Natural resources in the Nanortalik district. An interview study on fishing, hunting and tourism in the area around the Nalunaq gold project. Na- tional Environmental Research Institute, Technical Report No. 384: 81 pp. Reproduction is permitted, provided the source is explicitly acknowledged. Abstract: The interview study was performed in the Nanortalik municipality, South Green- land, during March-April 2001. It is a part of an environmental baseline study done in relation to the Nalunaq gold project. 23 fishermen, hunters and others gave infor- mation on 11 fish species, Snow crap, Deep-sea prawn, five seal species, Polar bear, Minke whale and two bird species; moreover on gathering of mussels, seaweed etc., sheep farms, tourist localities and areas for recreation. -
Sheep Farming As “An Arduous Livelihood”
University of Alberta Cultivating Place, Livelihood, and the Future: An Ethnography of Dwelling and Climate in Western Greenland by Naotaka Hayashi A thesis submitted to the Faculty of Graduate Studies and Research in partial fulfillment of the requirements for the degree of Doctor of Philosophy Department of Anthropology ©Naotaka Hayashi Spring 2013 Edmonton, Alberta Permission is hereby granted to the University of Alberta Libraries to reproduce single copies of this thesis and to lend or sell such copies for private, scholarly or scientific research purposes only. Where the thesis is converted to, or otherwise made available in digital form, the University of Alberta will advise potential users of the thesis of these terms. The author reserves all other publication and other rights in association with the copyright in the thesis and, except as herein before provided, neither the thesis nor any substantial portion thereof may be printed or otherwise reproduced in any material form whatsoever without the author's prior written permission. Abstract In order to investigate how Inuit Greenlanders in western Greenland are experiencing, responding to, and thinking about recent allegedly human-induced climate change, this dissertation ethnographically examines the lives of Greenlanders as well as Norse and Danes in the course of past historical natural climate cycles. My emphasis is on human endeavours to cultivate a future in the face of difficulties caused by climatic and environmental transformation. I recognize locals’ initiatives to carve out a future in the promotion of sheep farming and tree-planting in southern Greenland and in adaptation processes of northern Greenlandic hunters to the ever-shifting environment. -
5 General Background to Greenland and Project
Angus & Ross plc - Nalunaq Gold Mine: Environmental Impact Assessment – 20 th July 2009 5 General Background to Greenland and Project Location of Nalunaq Gold Mine Illustrated in Figure 5.1, Greenland, (Kalaallit Nunaat in Greenlandic, Grønland Figure 5.1 – Generalised Sketch-Map of Greenland in Danish), is an internally self-governing part of Denmark, being a vast island situated between the North Atlantic and Arctic Oceans. Although geographically and ethnically an Arctic island nation associated with the continent of North America, politically and historically Greenland is closely tied to Europe, specifically Denmark and Norway. Greenland lies mostly north of Section B Page 1 of 33 Angus & Ross plc - Nalunaq Gold Mine: Environmental Impact Assessment – 20 th July 2009 the Arctic Circle and is separated from the Canadian Arctic Archipelago, to the west, primarily by the Davis Strait and Baffin Bay, and from Iceland, to the east, by the Strait of Denmark. The largest island in the world that is not also considered a continent, Greenland has a maximum length, from its northernmost point on Cape Morris Jesup to Cape Farewell in the extreme south, of about 2,655 km (1,650 mi). The maximum distance from east to west is about 1,290 km (800 mi). The length of Greenland's coast, which is deeply indented with fiords, is estimated at 5,800 km (3,600 mi). The total area of Greenland is approximately 2,175,600 sq km (840,000 sq mi), of which about 84 per cent, or some 1,834,000 sq km (708,000 sq mi), is ice cap which can be up to 3kms in thickness. -
Greenland.Pdf
Greenland: Security Perspectives Jørgen Taagholt Jens Claus Hansen translated by Daniel Lufkin he Atlantic Treaty Association was established in 1950 as a group of former Opposition Members Tand politicians from parties backing NATO membership: Social Democrats, Conservative People’s Party and the Left Party. The goal of the Atlantic Treaty Association is to foster understanding of Danish mem- bership in NATO and to advocate political, economic and cultural cooperation among the member nations. Primary emphasis in the activities of the Atlantic Treaty Association is on: • Cooperation with the Baltic states and the Central and Eastern European nations • Activities with young people interested in Danish security policy • Seminars and study tours for teachers from high schools and other educational institutions • Publication of timely articles. In informational and educational activities, we em- phasize: • European security—including the expansion of the EU in depth and breadth; • The role of the United States in European security policy; • The work of NATO and the EU—looking into the future. The Atlantic Treaty Association is a private, voluntary organization whose work is financed from public and private funds. Greenland: Security Perspectives Jørgen Taagholt Jens Claus Hansen translated by Daniel Lufkin Translated from the Danish by Daniel Lufkin. This book may be cited as: Taagholt, Jørgen, and Hansen, Jens Claus. 2001. Greenland: Security Perspectives. Trans. Daniel Lufkin. Fairbanks, Alaska: Arctic Research Consortium of the United States. This report is published by ARCUS with funding provided by the National Science Foundation (NSF) Arctic Social Sciences Program under cooperative agreement OPP-9727899. Any opinions, findings, and conclusions or recommendations expressed in this material are those of the authors and do not necessarily reflect the views of the NSF. -
Tourism Experiences in the Peripheral North
Naalakkersuisut Government of Greenland INUSSUK • Arctic Research Journal 2 • 2011 Tourism Experiences in the Peripheral North Case Studies from Greenland Daniela Tommasini Naalakkersuisut Government of Greenland INUSSUK • Arctic Research Journal 2 • 2011 Tourism Experiences in the Peripheral North Case Studies from Greenland Daniela Tommasini TOURISM EXPERIENCES IN THE PERIPHERAL NORTH - CASE STUDIES FROM GREENLAND INUSSUK - Arctic Research Journal 2 - 2011 Copyright © Government of Greenland, Ministry of Education & Research, Nuuk, 2011 Layout, maps and graphics: allu design - www.allu.gl Typeface: Arial Publisher: Ministry of Education & Research Print: AKA Print A/S, Århus 1. edition Print run: 500 copies ISBN 978-87-92554-34-5 ISSN 1397-7431 INUSSUK - Arctic Research Journal is published by the Ministry of Education & Research. Permission to publish extracts, including figures, tables and quotations, is granted as long as the source is clearly given. Copies are kindly requested of all works that refer to, announce, quote or make reference to this publication. It is the purpose of this journal to disseminate results of research in Arctic regions to the population of Greenland as well as research communities in Greenland and Denmark. The journal wishes to contribute to strengthening co-operation in Arctic research, in particular within the humanities, social sciences and public health. The editorial board welcomes proposals for publications. Editors Research Coordinator Najâraq Paniula Research Coordinator Lone Nukaaraq Møller Government of Greenland Government of Greenland Ministry of Education & Research Ministry of Education & Research P.O. Box 1029 P.O. Box 1029 3900 Nuuk, Greenland 3900 Nuuk, Greenland Telephon: +299 34 50 00 Telephon: +299 34 50 00 Fax: +299 32 20 73 Fax: +299 32 20 73 E-mail: [email protected] E-mail: [email protected] www.nanoq.gl www.nanoq.gl Publications in the series can be ordered from: Ministry of Education & Research P.O. -
Exploring Greenland
EDITED BY RONALD E. DOEL, KRISTINE C. HARPER & MATTHIAS HEYMANN EXPLORING GREENLAND Cold War Science and Technology on Ice PALGRAVE STUDIES IN THE HISTORY OF SCIENCE AND TECHNOLOGY Palgrave Studies in the History of Science and Technology Series Editors James Rodger Fleming Colby College Waterville , Maine , USA Roger D. Launius National Air and Space Museum Washington, D.C. , USA Designed to bridge the gap between the history of science and the history of technology, this series publishes the best new work by promising and accomplished authors in both areas. In particular, it offers historical per- spectives on issues of current and ongoing concern, provides international and global perspectives on scientifi c issues, and encourages productive communication between historians and practicing scientists. More information about this series at http://www.springer.com/series/14581 Ronald E. Doel • Kristine C. Harper • Matthias Heymann Editors Exploring Greenland Cold War Science and Technology on Ice Editors Ronald E. Doel Matthias Heymann Florida State University Aarhus University Tallahassee , Florida , USA Aarhus , Denmark Kristine C. Harper Florida State University Tallahassee, Florida, USA Palgrave Studies in the History of Science and Technology ISBN 978-1-137-59687-1 ISBN 978-1-137-59688-8 (eBook) DOI 10.1057/978-1-137-59688-8 Library of Congress Control Number: 2016936665 © The Editor(s) (if applicable) and The Author(s) 2016 This work is subject to copyright. All rights are solely and exclusively licensed by the Publisher, whether the whole or part of the material is concerned, specifi cally the rights of translation, reprinting, reuse of illustrations, recitation, broadcasting, reproduction on microfi lms or in any other physical way, and transmission or information storage and retrieval, electronic adaptation, computer software, or by similar or dissimilar methodology now known or hereafter developed. -
A More Greenlandic Greenland
As of 2008 Museum Tusculanum Press has taken over the series Monographs on Greenland | Meddelelser om Grønland. Manuscripts should be sent to: Museum Tusculanum Press University of Copenhagen 126 Njalsgade, DK-2300 Copenhagen S DENMARK [email protected] | www.mtp.dk Tel. +45 353 29109 | Fax +45 353 29113 VAT no.: 8876 8418 Guidelines for authors can be found at www.mtp.dk/MoG Orders Books can be purchased online at www.mtp.dk, via [email protected], through any of MTP’s distributors in the US, UK, and France or via online retailers and major booksellers. Museum Tusculanum Press bank details: Amagerbanken, DK-2300 Copenhagen S :: BIC: AMBK DK KK :: IBAN: DK10 5202 0001 5151 08 More information at www.mtp.dk/MoG About the series Monographs on Greenland | Meddelelser om Grønland (ISSN 0025 6676) has published scientific results from all fields of research on Greenland since 1878. The series numbers more than 345 volumes comprising more than 1250 titles. In 1979 Monographs on Greenland | Meddelelser om Grønland was developed into a tripartite series consisting of Bioscience (ISSN 0106-1054) Man & Society (ISSN 0106-1062) Geoscience (ISSN 0106-1046) Hence Monographs on Greenland | Meddelelser om Grønland was renumbered in 1979 ending with volume no. 206 and continued with volume no. 1 for each subseries. As of 2008 the original Monographs on Greenland | Meddelelser om Grønland numbering will be continued in addition to the subseries numbering. Further information about the series, including addresses of the scientific editors of the subseries can be found at www.mtp.dk/MoG Denmark-Greenland in the twentieth Century Denmark-Greenland in the twentieth Century Axel Kjær Sørensen Meddelelser om Grønland · Man & Society 34 Axel Kjær Sørensen: Denmark – Greenland in the twentieth Century. -
Adobe PDF of Proposed Flight Lines
Spring 2015 IceBridge WP-3D Flight Plans 11 March 2016 Draft compiled by John Sonntag Introduction to Flight Plans This document is a translation of the NASA Operation IceBridge (OIB) scientific objectives articulated in the Level 1 OIB Science Requirements, at the January IceBridge Arctic planning meeting held at NASA GSFC, through official science team telecons and through e-mail communication and iterations into a series of operationally realistic flight plans, intended to be flown aboard NOAA's WP-3D aircraft, beginning in late-March and ending in mid-May 2016. The material is shown on the following pages in the distilled form of a map and brief text description of each science flight. Google Earth (KML) versions of these flight plans are available via anonymous FTP at the following address: ftp://atm.wff.nasa.gov/outgoing/oibscienceteam/. Note that some users have reported problems connecting to this address with certain browsers. Command-line FTP and software tools such as Filezilla may be of help in such situations. For each planned mission, we give a map and brief text description for the mission. The missions are planned to be flown from Thule and Kangerlussuaq, Greenland, and Fairbanks, Alaska. A careful reader may notice that some of the mission maps in the main part of the document highlight flightlines in green, yellow, and red colors, while other only show the black lines. The colors are a refinement added to the flight plans at a late stage of design which help the field team navigate the aircraft properly to achieve specific science goals. -
The Distributio of 10 Meter Snow Temperatures on the Greenland Ice Sheet
THE DISTRIBUTIO OF 10 METER SNOW TEMPERATURES ON THE GREENLAND ICE SHEET By S. J. MOCK and W. F. WEEKS (U.S. Army Cold Regions Research and Engineering Laboratory, Hanover, New Hampshire, U .S.A. ) ABSTRACT. A ll available 10 m. snow temperatures from the Greenland Ice Sheet have been analyzed using multiple regression techniques to develop equations capable of accurately predicting these temperatures. The analysis was carri ed out for all Greenland and for various sub-areas. The resulting equations show that 10 m . snow temperatures can be accurately predicted from the parameters latitude and elevation. Longitude was found to be a further significant parameter in south Greenland. Gradients of 10 m. snow temperatures versus elevation for north Greenland are close to the dry adiabatic lapse rate indicating adiabatic warming of katabatic w inds as the controlling mechanism in their a ltitudina l distribution. In both south Greenland and the Thule peninsula , gradients of 10 m. snow temperatures versus elevation are markedly greater than the dry adiabatic lapse rate and are highly dependent upon elevation, indicating downward transfer of latent heat in the snow, largely as a res ult of percolating melt water. An isotherm map, showing the distribution of 10 m. snow temperatures on the Greenland Ice Sheet calcula ted from the prediction equations, was preparecl. The map is based upon a revised conto ur map of the ice sheet made from a compilation of a ll known elevations. R EsUME. Dislribulion des lemJHfralllres a 1 0 melres de pro.fondenr de la neige de l'illdlandsis d" Groenland. -
Supplementary Material For: Evaluation of Greenland Near Surface Air Temperature Datasets J
Supplementary material for: Evaluation of Greenland near surface air temperature datasets J. E. Jack Reeves Eyre1 and Xubin Zeng1 1Department of Hydrology and Atmospheric Sciences, University of Arizona, Tucson, 85721, USA 5 Correspondence to: J. E. Jack Reeves Eyre ([email protected]) 1 Figure S1: Mean over station-months of bias (a, c, e) and absolute error (b, d, f) relative to monthly mean SAT at: ice sheet stations above 1500 m (a and b); ice sheet stations below 1500 m (c and d); and coastal (DMI) stations (e and f). Ice sheet stations 5 are from GC-Net, PROMICE and K-transect. All available station months from 1979 onwards are used. This figure is the same as Fig. 4 except that elevation corrections are not applied to any datasets. 2 Figure S2: As Fig. S1 but for the two longer reanalyses and MAR-20CR. 3 Table S1: Names and locations of weather stations used in this study. The DMI stations shown here represent the compilation of multiple stations at slightly different locations, with differing names (e.g., the Danish name for Ittoqqortoormiit is Scoresbysund). See Cappelen (2013) for more details. Station name Latitude Longitude Elevation Station name Latitude Longitude Elevation (North) (West) / m (North) (West) / m Coastal DMI Danmarkshavn 76.77 18.67 11 Nuuk 64.18 51.73 80 Ittoqqortoormiit 70.48 21.95 70 Pituffik 76.53 68.75 77 Ilulissat 69.24 51.07 29 Qaqortoq 60.72 46.05 57 Ivittuut 61.20 48.18 30 Tasiilaq 65.61 37.64 54 Narsarsuaq 61.17 45.42 34 Upernavik 72.78 56.13 126 Ice sheet GC-Net Swiss Camp 69.56 49.33 1176 -
Satellite Image Atlas of Glaciers of the World -- Greenland
Satellite Image Atlas of Glaciers of the World GREENLAND United States Geological Survey Professional Paper 1386-C Cover: Landsat 1 MSS digitally enhanced false-color composite image of the south slope of the Inland Ice northwest of Julianehåb (Qaqortoq), South Greenland. The image shows the well-defined edge of the Inland Ice, which spreads over the lowlands of South Greenland toward the outer coast. In some areas, the snowline is clearly visible. The marginal areas of the ice sheet in this area have undergone strong thinning during this century. (Landsat image 22061-13461, 13 September 1980; Path 3, Row 17 from Canada Centre for Remote Sensing, Ottawa, Ontario.) GREENLAND By ANKER WEIDICK With a section on LANDSAT IMAGES OF GREENLAND By RICHARD S. WILLIAMS, Jr., and JANE G. FERRIGNO SATELLITE IMAGE ATLAS OF GLACIERS OF THE WORLD Edited by RICHARD S. WILLIAMS, Jr., and JANE G. FERRIGNO U.S. GEOLOGICAL SURVEY PROFESSIONAL PAPER 1386-C Landsat images are the basis for a discussion of the glaciology, geography, and climatology of Greenland, the second largest areal and volumetric concentration of present-day glacier ice UNITED STATES GOVERNMENT PRINTING OFFICE, WASHINGTON: 1995 U.S. DEPARTMENT OF THE INTERIOR BRUCE BABBITT, Secretary U.S. GEOLOGICAL SURVEY Gordon P. Eaton, Director Any use of trade, product, orfirm names in this publication is for descriptive purposes only and does not imply endorsement by the U.S. Government Technical editing by John M. Watson Design and illustrations by Lynn Hulett Graphics Team: Arlene Compher, Maura Hogan, Dave Murphy, and Jane Russell Typesetting by Carolyn McQuaig Library of Congress Cataloging in Publication Data (Revised for vol. -
Supplement of the Cryosphere, 11, 1591–1605, 2017 © Author(S) 2017
Supplement of The Cryosphere, 11, 1591–1605, 2017 https://doi.org/10.5194/tc-11-1591-2017-supplement © Author(s) 2017. This work is distributed under the Creative Commons Attribution 3.0 License. Supplement of Evaluation of Greenland near surface air temperature datasets J. E. J. Reeves Eyre and X. Zeng Correspondence to: J. E. Jack Reeves Eyre ([email protected]) The copyright of individual parts of the supplement might differ from the CC BY 3.0 License. Figure S1. SAT time series from six stations on the ice sheet for 2010 and 2011: (a) Summit (GC–Net) is at ~3200 m, close to the topographic summit of the ice sheet; (b) station S9 (K–transect) is at 1500m on the western flank of the ice sheet; (c) station KAN_M (PROMICE) is at 1270 m, close to the K-transect stations; (d) Swiss Camp (GC–Net) is at ~1200 m, north of the K– 5 transect; (e) KPC_U (PROMICE) is at 870 m in the north-east of the ice sheet; (f) TAS_U (PROMICE) is at 570 m in the south- east of the ice sheet. 2 Figure S2: Mean over station-months of bias (a, c, e) and absolute error (b, d, f) relative to monthly mean SAT at: ice sheet stations above 1500 m (a and b); ice sheet stations below 1500 m (c and d); and coastal (DMI) stations (e and f). Ice sheet stations are from GC–Net, PROMICE and K–transect. All available station months from 1979 onwards are used. This figure is the same as 5 Fig.