Full Waveform Tomographic Images of the Peak Ring at the Chicxulub Impact Crater J
Total Page:16
File Type:pdf, Size:1020Kb
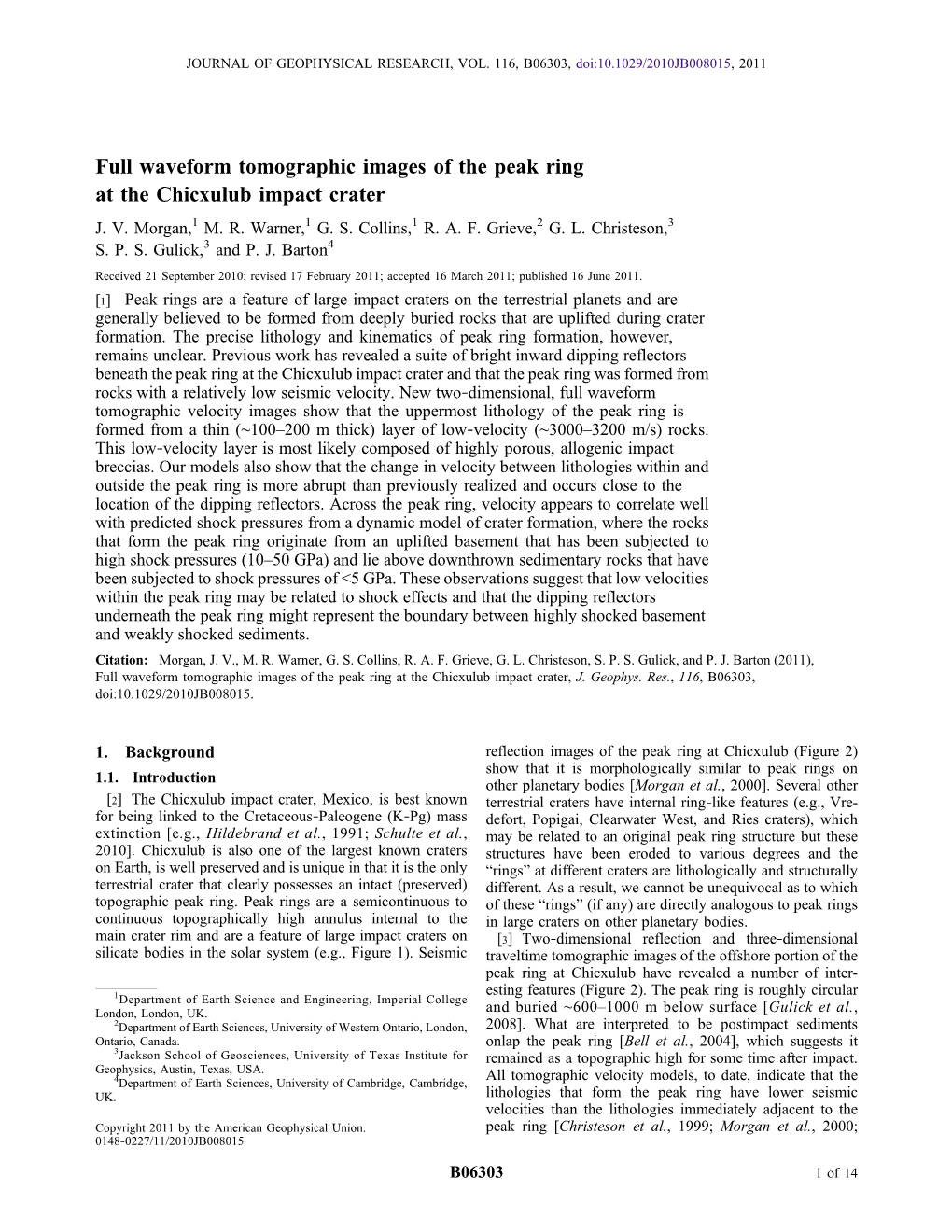
Load more
Recommended publications
-
Extraordinary Rocks from the Peak Ring of the Chicxulub Impact Crater: P-Wave Velocity, Density, and Porosity Measurements from IODP/ICDP Expedition 364 ∗ G.L
Earth and Planetary Science Letters 495 (2018) 1–11 Contents lists available at ScienceDirect Earth and Planetary Science Letters www.elsevier.com/locate/epsl Extraordinary rocks from the peak ring of the Chicxulub impact crater: P-wave velocity, density, and porosity measurements from IODP/ICDP Expedition 364 ∗ G.L. Christeson a, , S.P.S. Gulick a,b, J.V. Morgan c, C. Gebhardt d, D.A. Kring e, E. Le Ber f, J. Lofi g, C. Nixon h, M. Poelchau i, A.S.P. Rae c, M. Rebolledo-Vieyra j, U. Riller k, D.R. Schmitt h,1, A. Wittmann l, T.J. Bralower m, E. Chenot n, P. Claeys o, C.S. Cockell p, M.J.L. Coolen q, L. Ferrière r, S. Green s, K. Goto t, H. Jones m, C.M. Lowery a, C. Mellett u, R. Ocampo-Torres v, L. Perez-Cruz w, A.E. Pickersgill x,y, C. Rasmussen z,2, H. Sato aa,3, J. Smit ab, S.M. Tikoo ac, N. Tomioka ad, J. Urrutia-Fucugauchi w, M.T. Whalen ae, L. Xiao af, K.E. Yamaguchi ag,ah a University of Texas Institute for Geophysics, Jackson School of Geosciences, Austin, USA b Department of Geological Sciences, Jackson School of Geosciences, Austin, USA c Department of Earth Science and Engineering, Imperial College, London, UK d Alfred Wegener Institute Helmholtz Centre of Polar and Marine Research, Bremerhaven, Germany e Lunar and Planetary Institute, Houston, USA f Department of Geology, University of Leicester, UK g Géosciences Montpellier, Université de Montpellier, France h Department of Physics, University of Alberta, Canada i Department of Geology, University of Freiburg, Germany j SM 312, Mza 7, Chipre 5, Resid. -
Pro.Ffress D &Dentsdc Raddo
Pro.ffress d_ &dentSdc Raddo FIFTEENTH GENERAL ASSEMBLY OF THE INTERNATIONAL SCIENTIFIC RADIO UNION September 5-15, 1966 Munich, Germany REPORT OF THE U.S.A. NATIONAL COMMITTEE OF THE INTERNATIONAL SCIENTIFIC RADIO UNION Publication 1468 NATIONAL ACADEMY OF SCIENCES NATIONAL RESEARCH COUNCIL Washington, D.C. 1966 Library of Congress Catalog Card No. 55-31605 Available from Printing and Publishing Office National Academy of Sciences 2101 Constitution Avenue Washington, D.C. 20418 Price: $10.00 October28,1966 Dear Dr. Seitz: I ampleasedto transmitherewitha full report to the NationalAcademy of Sciences--NationalResearchCouncil,onthe 15thGeneralAssemblyof URSIwhichwasheldin Munich,September5-15, 1966. TheUnitedStatesNationalCommitteeof URSIhasparticipatedin the affairs of the Unionfor forty-five years. It hashadmuchinfluenceonthe Unionas,.for example,in therecent creationof a Commissiononthe Mag- netosphere.ThisnewCommission,whichis nowvery strong,demonstrates the ability of theUnion,oneof ICSU'sthree oldest,to respondto the changingneedsof its field. AlthoughtheUnitedStatessendsthelargest delegationsof anycountry to the GeneralAssembliesof URSI,theyare neverthelessrelatively small becauseof thestrict mannerin whichURSIcontrolsthe size of its Assem- blies. This is donein order to preventineffectivenessthroughuncontrolled participationbyvery largenumbersof delegates.Accordingly,our delega- tions are carefully selected,andcomprisepeoplequalifiedto prepareand presentour NationalReportto the Assembly.Thepre-Assemblyreport is a report of progressin -
Impact Cratering
6 Impact cratering The dominant surface features of the Moon are approximately circular depressions, which may be designated by the general term craters … Solution of the origin of the lunar craters is fundamental to the unravel- ing of the history of the Moon and may shed much light on the history of the terrestrial planets as well. E. M. Shoemaker (1962) Impact craters are the dominant landform on the surface of the Moon, Mercury, and many satellites of the giant planets in the outer Solar System. The southern hemisphere of Mars is heavily affected by impact cratering. From a planetary perspective, the rarity or absence of impact craters on a planet’s surface is the exceptional state, one that needs further explanation, such as on the Earth, Io, or Europa. The process of impact cratering has touched every aspect of planetary evolution, from planetary accretion out of dust or planetesimals, to the course of biological evolution. The importance of impact cratering has been recognized only recently. E. M. Shoemaker (1928–1997), a geologist, was one of the irst to recognize the importance of this process and a major contributor to its elucidation. A few older geologists still resist the notion that important changes in the Earth’s structure and history are the consequences of extraterres- trial impact events. The decades of lunar and planetary exploration since 1970 have, how- ever, brought a new perspective into view, one in which it is clear that high-velocity impacts have, at one time or another, affected nearly every atom that is part of our planetary system. -
Mighty Eagle: the Development and Flight Testing of an Autonomous Robotic Lander Test Bed
Mighty Eagle: The Development and Flight Testing of an Autonomous Robotic Lander Test Bed Timothy G. McGee, David A. Artis, Timothy J. Cole, Douglas A. Eng, Cheryl L. B. Reed, Michael R. Hannan, D. Greg Chavers, Logan D. Kennedy, Joshua M. Moore, and Cynthia D. Stemple PL and the Marshall Space Flight Center have been work- ing together since 2005 to develop technologies and mission concepts for a new generation of small, versa- tile robotic landers to land on airless bodies, including the moon and asteroids, in our solar system. As part of this larger effort, APL and the Marshall Space Flight Center worked with the Von Braun Center for Science and Innovation to construct a prototype monopropellant-fueled robotic lander that has been given the name Mighty Eagle. This article provides an overview of the lander’s architecture; describes the guidance, navi- gation, and control system that was developed at APL; and summarizes the flight test program of this autonomous vehicle. INTRODUCTION/PROJECT BACKGROUND APL and the Marshall Space Flight Center (MSFC) technology risk-reduction efforts, illustrated in Fig. 1, have been working together since 2005 to develop have been performed to explore technologies to enable technologies and mission concepts for a new genera- low-cost missions. tion of small, autonomous robotic landers to land on As part of this larger effort, MSFC and APL also airless bodies, including the moon and asteroids, in our worked with the Von Braun Center for Science and solar system.1–9 This risk-reduction effort is part of the Innovation (VCSI) and several subcontractors to con- Robotic Lunar Lander Development Project (RLLDP) struct the Mighty Eagle, a prototype monopropellant- that is directed by NASA’s Planetary Science Division, fueled robotic lander. -
The Curtis L. Ivey Science Center DEDICATED SEPTEMBER 17, 2004
NON-PROFIT Office of Advancement ORGANIZATION ALUMNI MAGAZINE COLBY-SAWYER Colby-Sawyer College U.S. POSTAGE 541 Main Street PAID New London, NH 03257 LEWISTON, ME PERMIT 82 C LBY-SAWYER CHANGE SERVICE REQUESTED ALUMNI MAGAZINE I NSIDE: FALL/WINTER 2004 The Curtis L. Ivey Science Center DEDICATED SEPTEMBER 17, 2004 F ALL/WINTER 2004 Annual Report Issue EDITOR BOARD OF TRUSTEES David R. Morcom Anne Winton Black ’73, ’75 CLASS NOTES EDITORS Chair Tracey Austin Ye ar of Gaye LaCasce Philip H. Jordan Jr. Vice-Chair CONTRIBUTING WRITERS Tracey Austin Robin L. Mead ’72 the Arts Jeremiah Chila ’04 Executive Secretary Cathy DeShano Ye ar of Nicole Eaton ’06 William S. Berger Donald A. Hasseltine Pamela Stanley Bright ’61 Adam S. Kamras Alice W. Brown Gaye LaCasce Lo-Yi Chan his month marks the launch of the Year of the Arts, a David R. Morcom Timothy C. Coughlin P’00 Tmultifaceted initiative that will bring arts faculty members to meet Kimberly Swick Slover Peter D. Danforth P’83, ’84, GP’02 the Arts Leslie Wright Dow ’57 with groups of alumni and friends around the country. We will host VICE PRESIDENT FOR ADVANCEMENT Stephen W. Ensign gatherings in art museums and galleries in a variety of cities, and Donald A. Hasseltine Eleanor Morrison Goldthwait ’51 are looking forward to engaging hundreds of alumni and friends in Suzanne Simons Hammond ’66 conversations about art, which will be led by our faculty experts. DIRECTOR OF DEVELOPMENT Patricia Driggs Kelsey We also look forward to sharing information about Colby-Sawyer’s Beth Cahill Joyce Juskalian Kolligian ’55 robust arts curriculum. -
Appendix I Lunar and Martian Nomenclature
APPENDIX I LUNAR AND MARTIAN NOMENCLATURE LUNAR AND MARTIAN NOMENCLATURE A large number of names of craters and other features on the Moon and Mars, were accepted by the IAU General Assemblies X (Moscow, 1958), XI (Berkeley, 1961), XII (Hamburg, 1964), XIV (Brighton, 1970), and XV (Sydney, 1973). The names were suggested by the appropriate IAU Commissions (16 and 17). In particular the Lunar names accepted at the XIVth and XVth General Assemblies were recommended by the 'Working Group on Lunar Nomenclature' under the Chairmanship of Dr D. H. Menzel. The Martian names were suggested by the 'Working Group on Martian Nomenclature' under the Chairmanship of Dr G. de Vaucouleurs. At the XVth General Assembly a new 'Working Group on Planetary System Nomenclature' was formed (Chairman: Dr P. M. Millman) comprising various Task Groups, one for each particular subject. For further references see: [AU Trans. X, 259-263, 1960; XIB, 236-238, 1962; Xlffi, 203-204, 1966; xnffi, 99-105, 1968; XIVB, 63, 129, 139, 1971; Space Sci. Rev. 12, 136-186, 1971. Because at the recent General Assemblies some small changes, or corrections, were made, the complete list of Lunar and Martian Topographic Features is published here. Table 1 Lunar Craters Abbe 58S,174E Balboa 19N,83W Abbot 6N,55E Baldet 54S, 151W Abel 34S,85E Balmer 20S,70E Abul Wafa 2N,ll7E Banachiewicz 5N,80E Adams 32S,69E Banting 26N,16E Aitken 17S,173E Barbier 248, 158E AI-Biruni 18N,93E Barnard 30S,86E Alden 24S, lllE Barringer 29S,151W Aldrin I.4N,22.1E Bartels 24N,90W Alekhin 68S,131W Becquerei -
Pirstekartioista Keurusselällä Ja Maailmalla TEEMU ÖHMAN
Pirstekartioista Keurusselällä ja maailmalla TEEMU ÖHMAN li erittäin ilahduttavaa huomata, että kittävä vaikutus jatkotutkimusten ohjaamisessa lu- jo Marmon (1963, s. 16) mitä ilmei- paavimpiin kohteisiin. Geofysiikalla ei kuitenkaan simmin kuvaamia, joskaan ei tör- koskaan todisteta mahdollisen kraatterin synnystä mäyssyntyisiksi tulkitsemia Keurus- tai pirstekartioista sitä eikä tätä. Se, että törmäys- Oselän pirstekartioita on intouduttu tutkimaan mi- malli selittää muutoin käsittämättömiksi jäävät neralogisesta näkökulmasta (Kinnunen ja Hietala havainnot, joita “perinteisin” teorioin on turhaan 2009, jatkossa KH09). Myös mielipiteiden nopea yritetty ymmärtää, usein vuosikymmenien ajan, on vaihtuminen (KH09 vs. esimerkiksi Hietala ja Moi- varsin vahva viite mallin oikeellisuuden puolesta, lanen 2004, 2007, Pesonen et al . 2005, Ruotsalai- eikä suinkaan kerro siitä, etteikö muita selitysmal- nen et al . 2006 ja vielä Schmieder et al . 2009) on leja olisi yritetty näihin kohteisiin soveltaa. virkistävä poikkeus usein hyvin jääräpäisessä geo- KH09:n väite, että kiistattomia todisteita tör- tieteellisessä tutkimuksessa. Kuten KH09 toteavat- mäyksistä löydettäisiin vain “geologisesti melko kin, ei pirstekartioiden mineralogiaan ole maail- nuorista” kraattereista, on yksinkertaisesti väärä, mallakaan järin paljon huomiota kiinnitetty, ja pirs- ellei sitten lähdetä tieteenfilosofiseen hetteikköön tekartioiden synty on useammastakin teoriasta pohtimaan sitä, mikä on kiistaton todiste. Pääsään- huolimatta edelleen hämärän peitossa. KH09 esit- -
Impact Structures and Events – a Nordic Perspective
107 by Henning Dypvik1, Jüri Plado2, Claus Heinberg3, Eckart Håkansson4, Lauri J. Pesonen5, Birger Schmitz6, and Selen Raiskila5 Impact structures and events – a Nordic perspective 1 Department of Geosciences, University of Oslo, P.O. Box 1047, Blindern, NO 0316 Oslo, Norway. E-mail: [email protected] 2 Department of Geology, University of Tartu, Vanemuise 46, 51014 Tartu, Estonia. 3 Department of Environmental, Social and Spatial Change, Roskilde University, P.O. Box 260, DK-4000 Roskilde, Denmark. 4 Department of Geography and Geology, University of Copenhagen, Øster Voldgade 10, DK-1350 Copenhagen, Denmark. 5 Division of Geophysics, University of Helsinki, P.O. Box 64, FIN-00014 Helsinki, Finland. 6 Department of Geology, University of Lund, Sölvegatan 12, SE-22362 Lund, Sweden. Impact cratering is one of the fundamental processes in are the main reason that the Nordic countries are generally well- the formation of the Earth and our planetary system, as mapped. reflected, for example in the surfaces of Mars and the Impact craters came into the focus about 20 years ago and the interest among the Nordic communities has increased during recent Moon. The Earth has been covered by a comparable years. The small Kaalijärv structure of Estonia was the first impact number of impact scars, but due to active geological structure to be confirmed in northern Europe (Table 1; Figures 1 and processes, weathering, sea floor spreading etc, the num- 7). First described in 1794 (Rauch), the meteorite origin of the crater ber of preserved and recognized impact craters on the field (presently 9 craters) was proposed much later in 1919 (Kalju- Earth are limited. -
Apollo 17 Index: 70 Mm, 35 Mm, and 16 Mm Photographs
General Disclaimer One or more of the Following Statements may affect this Document This document has been reproduced from the best copy furnished by the organizational source. It is being released in the interest of making available as much information as possible. This document may contain data, which exceeds the sheet parameters. It was furnished in this condition by the organizational source and is the best copy available. This document may contain tone-on-tone or color graphs, charts and/or pictures, which have been reproduced in black and white. This document is paginated as submitted by the original source. Portions of this document are not fully legible due to the historical nature of some of the material. However, it is the best reproduction available from the original submission. Produced by the NASA Center for Aerospace Information (CASI) Preparation, Scanning, Editing, and Conversion to Adobe Portable Document Format (PDF) by: Ronald A. Wells University of California Berkeley, CA 94720 May 2000 A P O L L O 1 7 I N D E X 7 0 m m, 3 5 m m, A N D 1 6 m m P H O T O G R A P H S M a p p i n g S c i e n c e s B r a n c h N a t i o n a l A e r o n a u t i c s a n d S p a c e A d m i n i s t r a t i o n J o h n s o n S p a c e C e n t e r H o u s t o n, T e x a s APPROVED: Michael C . -
Appendix a Recovery of Ejecta Material from Confirmed, Probable
Appendix A Recovery of Ejecta Material from Confirmed, Probable, or Possible Distal Ejecta Layers A.1 Introduction In this appendix we discuss the methods that we have used to recover and study ejecta found in various types of sediment and rock. The processes used to recover ejecta material vary with the degree of lithification. We thus discuss sample processing for unconsolidated, semiconsolidated, and consolidated material separately. The type of sediment or rock is also important as, for example, carbonate sediment or rock is processed differently from siliciclastic sediment or rock. The methods used to take and process samples will also vary according to the objectives of the study and the background of the investigator. We summarize below the methods that we have found useful in our studies of distal impact ejecta layers for those who are just beginning such studies. One of the authors (BPG) was trained as a marine geologist and the other (BMS) as a hard rock geologist. Our approaches to processing and studying impact ejecta differ accordingly. The methods used to recover ejecta from unconsolidated sediments have been successfully employed by BPG for more than 40 years. A.2 Taking and Handling Samples A.2.1 Introduction The size, number, and type of samples will depend on the objective of the study and nature of the sediment/rock, but there a few guidelines that should be followed regardless of the objective or rock type. All outcrops, especially those near industrialized areas or transportation routes (e.g., highways, train tracks) need to be cleaned off (i.e., the surface layer removed) prior to sampling. -
Chicxulub and the Exploration of Large Peak- Ring Impact Craters Through Scientific Drilling
Chicxulub and the Exploration of Large Peak- Ring Impact Craters through Scientific Drilling David A. Kring, Lunar and Planetary Institute, Houston, Texas 77058, USA; Philippe Claeys, Analytical, Environmental and Geo-Chemistry, Vrije Universiteit Brussel, Pleinlaan 2, Brussels 1050, Belgium; Sean P.S. Gulick, Institute for Geophysics and Dept. of Geological Sciences, Jackson School of Geosciences, University of Texas at Austin, Austin, Texas 78758, USA; Joanna V. Morgan and Gareth S. Collins, Dept. of Earth Science and Engineering, Imperial College London SW7 2AZ, UK; and the IODP-ICDP Expedition 364 Science Party. ABSTRACT proving the structure had an impact origin. to assess the depth of origin of the peak- The Chicxulub crater is the only well- The buried structure was confirmed by ring rock types and determine how they preserved peak-ring crater on Earth and seismic surveys conducted in 1996 and were deformed during the crater-forming linked, famously, to the K-T or K-Pg mass 2005 to be a large ~180–200-km–diameter event. That information is needed to effec- impact crater with an intact peak ring tively test how peak-ring craters form on extinction event. For the first time, geolo- (Morgan et al., 1997; Gulick et al., 2008). planetary bodies. gists have drilled into the peak ring of that The discovery of the Chicxulub impact The expedition was also designed to crater in the International Ocean structure initially prompted two scientific measure any hydrothermal alteration in Discovery Program and International drilling campaigns. In the mid-1990s, a the peak ring and physical properties of the Continental Scientific Drilling Program series of shallow onshore wells up to 700 m rocks, such as porosity and permeability, (IODP-ICDP) Expedition 364. -
Apollo 17 Index
Preparation, Scanning, Editing, and Conversion to Adobe Portable Document Format (PDF) by: Ronald A. Wells University of California Berkeley, CA 94720 May 2000 A P O L L O 1 7 I N D E X 7 0 m m, 3 5 m m, A N D 1 6 m m P H O T O G R A P H S M a p p i n g S c i e n c e s B r a n c h N a t i o n a l A e r o n a u t i c s a n d S p a c e A d m i n i s t r a t i o n J o h n s o n S p a c e C e n t e r H o u s t o n, T e x a s APPROVED: Michael C . McEwen Lunar Screening and Indexing Group May 1974 PREFACE Indexing of Apollo 17 photographs was performed at the Defense Mapping Agency Aerospace Center under the direction of Charles Miller, NASA Program Manager, Aerospace Charting Branch. Editing was performed by Lockheed Electronics Company, Houston Aerospace Division, Image Analysis and Cartography Section, under the direction of F. W. Solomon, Chief. iii APOLLO 17 INDEX 70 mm, 35 mm, AND 16 mm PHOTOGRAPHS TABLE OF CONTENTS Page INTRODUCTION ................................................................................................................... 1 SOURCES OF INFORMATION .......................................................................................... 13 INDEX OF 16 mm FILM STRIPS ........................................................................................ 15 INDEX OF 70 mm AND 35 mm PHOTOGRAPHS Listed by NASA Photograph Number Magazine J, AS17–133–20193 to 20375.........................................