Electronic Reprint Binding of Gd3+ to the Neuronal Signalling Protein
Total Page:16
File Type:pdf, Size:1020Kb
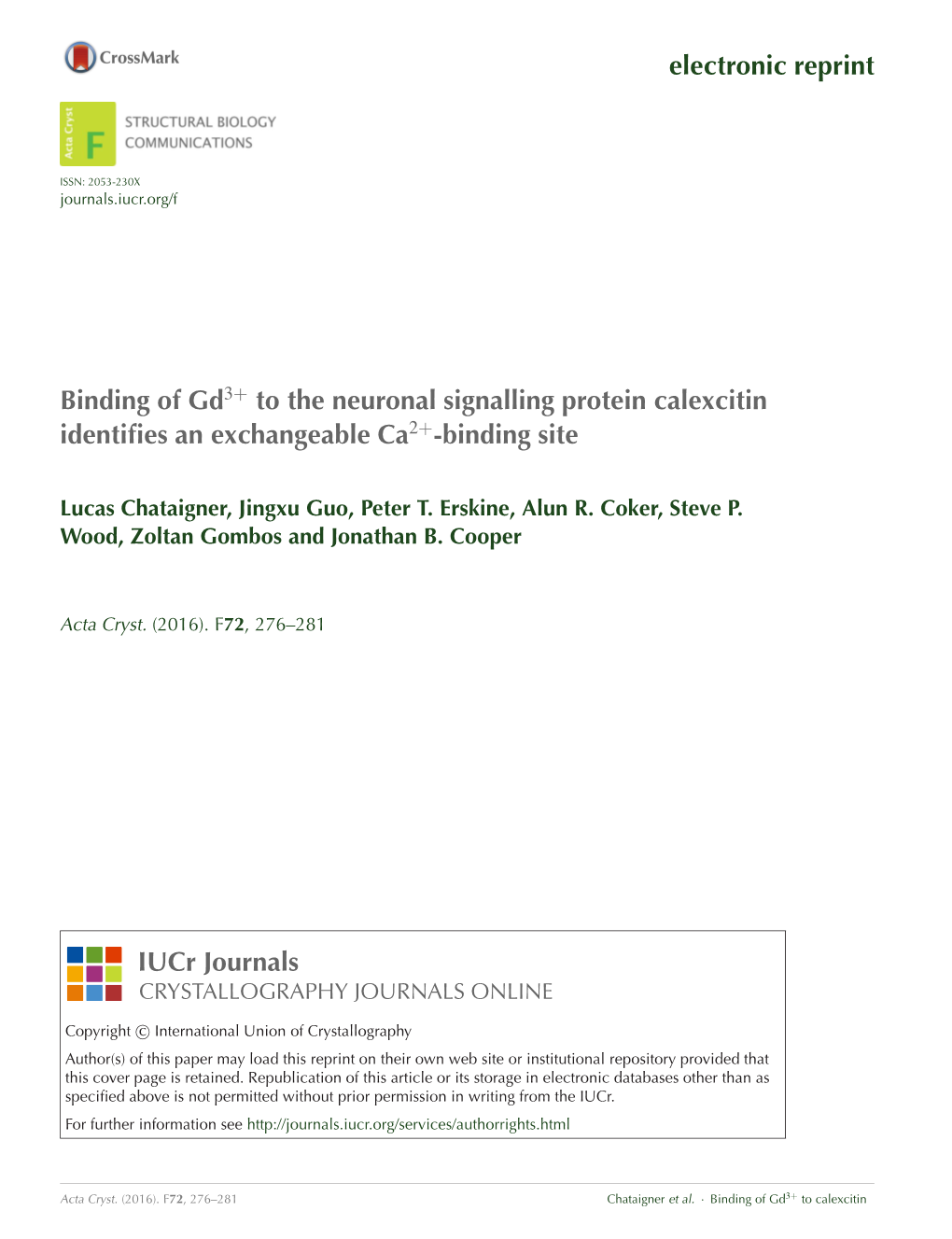
Load more
Recommended publications
-
Dependent Activation
Ca2؉-binding activity of a COOH-terminal fragment of the Drosophila BK channel involved in Ca2؉-dependent activation Shumin Bian*, Isabelle Favre*†, and Edward Moczydlowski*‡§ Departments of *Pharmacology and ‡Cellular and Molecular Physiology, Yale University School of Medicine, 333 Cedar Street, New Haven, CT 06520-8066 Communicated by Ramon Latorre, Center for Scientific Studies of Santiago, Valdivia, Chile, February 13, 2001 (received for review November 28, 2000) Mutational and biophysical analysis suggests that an intracellular S0–S6 plus a portion of the COOH terminus, whereas the Ϸ400 .(COOH-terminal domain of the large conductance Ca2؉-activated residue sequence following the linker comprises the Tail (Fig. 1 K؉ channel (BK channel) contains Ca2؉-binding site(s) that are Experiments with chimeric Core-Tail constructs by using Slo1 allosterically coupled to channel opening. However the structural genes of different species suggest that the Ca2ϩ-sensing function basis of Ca2؉ binding to BK channels is unknown. To pursue this of the channel is associated with the Tail domain (9). More question, we overexpressed the COOH-terminal 280 residues of the recent studies making use of the Ca2ϩ-insensitive Slo3 Kϩ Drosophila slowpoke BK channel (Dslo-C280) as a FLAG- and His6- channel gene verified that specific regions of protein sequence tagged protein in Escherichia coli. We purified Dslo-C280 in soluble within the Tail confer the property of Ca2ϩ-dependent gating ؉ ؉ form and used a 45Ca2 -overlay protein blot assay to detect Ca2 (10). Complementary mutational studies identified an Asp-rich ؉ binding. Dslo-C280 exhibits specific binding of 45Ca2 in compari- sequence motif in the Tail domain (Fig. -
Bryostatin Enhancement of Memory in Hermissenda
Reference: Biol. Bull. 210: 201–214. (June 2006) A contribution to The Biological Bulletin Virtual Symposium © 2006 Marine Biological Laboratory on Marine Invertebrate Models of Learning and Memory. Bryostatin Enhancement of Memory in Hermissenda A. M. KUZIRIAN1,*, H. T. EPSTEIN1, C. J. GAGLIARDI1,2, T. J. NELSON3, M. SAKAKIBARA4, C. TAYLOR5, A. B. SCIOLETTI1,6, AND D. L. ALKON3 1 Marine Biological Laboratory, Woods Hole, Massachusetts 02543; 2 Science Department, Roger Williams University, Bristol, Rhode Island 02809; 3 Blanchette Rockefeller Neurosciences Institute, Johns Hopkins University, Rockville, Maryland 20850; 4 Department of Biological Science and Technology, Tokai University, Shizuoka, Japan; 5 Department of Biology, University of Michigan, Ann Arbor, Michigan 48104; and 6 Northeastern University, Boston, Massachusetts 02115 Abstract. Bryostatin, a potent agonist of protein kinase C bryostatin revealed changes in input resistance and an en- (PKC), when administered to Hermissenda was found to hanced long-lasting depolarization (LLD) in response to affect acquisition of an associative learning paradigm. Low light. Likewise, quantitative immunocytochemical measure- bryostatin concentrations (0.1 to 0.5 ng/ml) enhanced mem- ments using an antibody specific for the PKC-activated ϩ ory acquisition, while concentrations higher than 1.0 ng/ml Ca2 /GTP-binding protein calexcitin showed enhanced an- down-regulated the pathway and no recall of the associative tibody labeling with bryostatin. training was exhibited. The extent of enhancement de- Animals exposed to the PKC inhibitor bisindolylmaleim- pended upon the conditioning regime used and the memory ide-XI (Ro-32-0432) administered by immersion prior to stage normally fostered by that regime. The effects of two 9-TE conditioning showed no training-induced changes training events (TEs) with paired conditioned and uncondi- with or without bryostatin exposure. -
Minding the Calcium Store: Ryanodine Receptor Activation As a Convergent Mechanism of PCB Toxicity
Pharmacology & Therapeutics 125 (2010) 260–285 Contents lists available at ScienceDirect Pharmacology & Therapeutics journal homepage: www.elsevier.com/locate/pharmthera Associate Editor: Carey Pope Minding the calcium store: Ryanodine receptor activation as a convergent mechanism of PCB toxicity Isaac N. Pessah ⁎, Gennady Cherednichenko, Pamela J. Lein Department of Molecular Biosciences, School of Veterinary Medicine, University of California, Davis, CA 95616, USA article info abstract Keywords: Chronic low-level polychlorinated biphenyl (PCB) exposures remain a significant public health concern since Ryanodine receptor (RyR) results from epidemiological studies indicate that PCB burden is associated with immune system Calcium-induced calcium release dysfunction, cardiovascular disease, and impairment of the developing nervous system. Of these various Calcium regulation adverse health effects, developmental neurotoxicity has emerged as a particularly vulnerable endpoint in Polychlorinated biphenyls PCB toxicity. Arguably the most pervasive biological effects of PCBs could be mediated by their ability to alter Triclosan fi 2+ Bastadins the spatial and temporal delity of Ca signals through one or more receptor-mediated processes. This Polybrominated diphenylethers review will focus on our current knowledge of the structure and function of ryanodine receptors (RyRs) in Developmental neurotoxicity muscle and nerve cells and how PCBs and related non-coplanar structures alter these functions. The Activity dependent plasticity molecular and cellular mechanisms by which non-coplanar PCBs and related structures alter local and global Ca2+ signaling properties and the possible short and long-term consequences of these perturbations on neurodevelopment and neurodegeneration are reviewed. © 2009 Elsevier Inc. All rights reserved. Contents 1. Introduction ............................................... 260 2. Ryanodine receptor macromolecular complexes: significance to polychlorinated biphenyl-mediated Ca2+ dysregulation . -
The Genie in the Bottle-Magnified Calcium Signaling in Dorsolateral
Molecular Psychiatry https://doi.org/10.1038/s41380-020-00973-3 EXPERT REVIEW The genie in the bottle-magnified calcium signaling in dorsolateral prefrontal cortex 1 1 1 Amy F. T. Arnsten ● Dibyadeep Datta ● Min Wang Received: 27 August 2020 / Revised: 20 November 2020 / Accepted: 26 November 2020 © The Author(s) 2020. This article is published with open access Abstract Neurons in the association cortices are particularly vulnerable in cognitive disorders such as schizophrenia and Alzheimer’s disease, while those in primary visual cortex remain relatively resilient. This review proposes that the special molecular mechanisms needed for higher cognitive operations confer vulnerability to dysfunction, atrophy, and neurodegeneration when regulation is lost due to genetic and/or environmental insults. Accumulating data suggest that higher cortical circuits rely on magnified levels of calcium (from NMDAR, calcium channels, and/or internal release from the smooth endoplasmic reticulum) near the postsynaptic density to promote the persistent firing needed to maintain, manipulate, and store information without “bottom-up” sensory stimulation. For example, dendritic spines in the primate dorsolateral prefrontal – – 1234567890();,: 1234567890();,: cortex (dlPFC) express the molecular machinery for feedforward, cAMP PKA calcium signaling. PKA can drive internal calcium release and promote calcium flow through NMDAR and calcium channels, while in turn, calcium activates adenylyl cyclases to produce more cAMP–PKA signaling. Excessive levels of cAMP–calcium signaling can have a number of detrimental effects: for example, opening nearby K+ channels to weaken synaptic efficacy and reduce neuronal firing, and over a longer timeframe, driving calcium overload of mitochondria to induce inflammation and dendritic atrophy. Thus, calcium–cAMP signaling must be tightly regulated, e.g., by agents that catabolize cAMP or inhibit its production (PDE4, mGluR3), and by proteins that bind calcium in the cytosol (calbindin). -
Crystallization and Preliminary X-Ray Diffraction Analysis of Calexcitin From
crystallization communications Acta Crystallographica Section F Structural Biology Crystallization and preliminary X-ray diffraction and Crystallization analysis of calexcitin from Loligo pealei: a neuronal Communications protein implicated in learning and memory ISSN 1744-3091 G. D. E. Beaven,a P. T. Erskine,a The neuronal protein calexcitin from the long-finned squid Loligo pealei has J. N. Wright,a F. Mohammed,a‡ been expressed in Escherichia coli and purified to homogeneity. Calexcitin is a R. Gill,a S. P. Wood,a J. Vernon,b 22 kDa calcium-binding protein that becomes up-regulated in invertebrates K. P. Gieseb and J. B. Coopera* following Pavlovian conditioning and is likely to be involved in signal transduction events associated with learning and memory. Recombinant squid aSchool of Biological Sciences, University of calexcitin has been crystallized using the hanging-drop vapour-diffusion Southampton, Bassett Crescent East, technique in the orthorhombic space group P212121. The unit-cell parameters Southampton SO16 7PX, England, and of a = 46.6, b = 69.2, c = 134.8 A˚ suggest that the crystals contain two monomers bWolfson Institute for Biomedical Research, per asymmetric unit and have a solvent content of 49%. This crystal form University College London, Cruciform Building, diffracts X-rays to at least 1.8 A˚ resolution and yields data of high quality using Gower Street, London WC1E 6BT, England synchrotron radiation. ‡ Present address: CRUK Institute for Cancer Studies, University of Birmingham, Vincent 1. Introduction Drive, Edgbaston, Birmingham B15 2TT, England. The protein calexcitin was originally identified in the photoreceptor neurons of the marine snail Hermissenda crassicornis as a 20 kDa protein that became up-regulated and phosphorylated following a Correspondence e-mail: Pavlovian training protocol (Nelson et al., 1990). -
Cell Biology of the Axon Editor Edward Koenig 70 Summer Hill Lane Williamsville NY 14221 USA [email protected]
Results and Problems in Cell Differentiation 48 Series Editors Dietmar Richter, Henri Tiedge Edward Koenig (ed.) Cell Biology of the Axon Editor Edward Koenig 70 Summer Hill Lane Williamsville NY 14221 USA [email protected] Series Editors Dietmar Richter Henri Tiedge Center for Molecular Neurobiology The Robert F. Furchgott Center for Neural and University Medical Center Hamburg- Behavioral Science Eppendorf (UKE) Department of Physiology and Pharmacology University of Hamburg Department of Neurology Martinistrasse 52 SUNY Health Science Center at Brooklyn 20246 Hamburg Brooklyn, New York 11203 Germany USA [email protected] [email protected] ISBN 978-3-642-03018-5 e-ISBN 978-3-642-03019-2 DOI 10.1007/978-3-642-03019-2 Springer Heidelberg Dordrecht London New York Results and Problems in Cell Differentiation ISSN 0080-1844 Library of Congress Control Number: 2009932721 © Springer-Verlag Berlin Heidelberg 2009 This work is subject to copyright. All rights are reserved, whether the whole or part of the material is concerned, specifically the rights of translation, reprinting, reuse of illustrations, recitation, broadcasting, reproduction on microfilm or in any other way, and storage in data banks. Duplication of this publication or parts thereof is permitted only under the provisions of the German Copyright Law of September 9, 1965, in its current version, and permission for use must always be obtained from Springer. Violations are liable for prosecution under the German Copyright Law. The use of registered names, trademarks, etc. in this publication does not imply, even in the absence of a specific statement, that such names are exempt from the relevant protective laws and regulations and therefore free for general use. -
Distinct Intracellular Calcium Transients in Neurites and Somata Integrate Neuronal Signals
The Journal of Neuroscience, July 1, 2002, 22(13):5344–5353 Distinct Intracellular Calcium Transients in Neurites and Somata Integrate Neuronal Signals Friedrich W. Johenning,1,3 Michal Zochowski,2 Stuart J. Conway,4 Andrew B. Holmes,4 Peter Koulen,1 and Barbara E. Ehrlich1,2 Departments of 1Pharmacology and 2Cellular and Molecular Physiology, Yale University, New Haven, Connecticut 06520, 3Department of Neuroanatomy, University Hospital Eppendorf, 20246 Hamburg, Germany, and 4Department of Chemistry, University of Cambridge, Cambridge CB2 1EW, United Kingdom Intracellular calcium signals have distinct temporal and spatial throughout the cell. In the soma, they serve as amplifying patterns in neurons in which signal initiation and repetitive molecular switches, facilitating recruitment of the InsP3R type spiking occurs predominantly in the neurite. We investigated III-dependent pool. In the neurite, they decrease the probability the functional implications of the coexpression of different iso- of repetitive spiking. Use of a cell-permeant analog of InsP3 forms of ryanodine receptors (RyR) and inositol 1,4,5- suggested that regional specificity in InsP3 production and trisphosphate receptors (InsP3Rs) using immunocytochemistry, surface-to-volume effects play minor roles in determining tem- Western blotting, and calcium imaging in neuronally differenti- poral and spatial calcium signaling patterns in neurons. Our ated PC12 cells. InsP3R type III, an isoform that has been findings suggest that additional modulatory processes acting shown to be upregulated in neuronal apoptosis, is exclusively on the intracellular channels are necessary to generate spatially expressed in the soma, serving as a gatekeeper for high- specific calcium signaling. magnitude calcium surges. InsP3R type I is expressed through- Key words: intracellular calcium signaling; inositol 1,4,5 out the cell and can be related to signal initiation and repetitive trisphosphate; InsP3 receptor; ryanodine receptor; PC12 cells; spiking in the neurite. -
Tertiary Alphabet for the Observable Protein Structural Universe”
Supporting Information for Mackenzie et al., “Tertiary Alphabet for the Observable Protein Structural Universe” SI Methods strongly correlate, resulting in shallow angles and large positive average cosines. Fig. S1 shows this general behavior, except that it also exhibits some oscillations RMSD Cutoff Derivation before it converges to zero. These oscillations have to do with a characteristic length of secondary structural elements (SSEs) separated by turns and loops, such Fundamentally, optimal rigid-body superposition is an instance of linear that within 15-30 residues a chain is expected to dramatically turn, often in regression, whose goal is to minimize the sum squared deviation (SSD) between almost the opposite direction. In fact, the overall curve fits very closely to a corresponding coordinates of two sets of atoms. Regression quality is typically product of exponential decay (due to the chain “forgetting” its direction) and a assessed using the mean squared error (MSE), an estimate of the error variance, sinusoid (from SSE-related oscillations), as shown in Fig. S1. Specifically, the computed as SSD �, where � is the number of degrees of freedom. The RMSD equation we used was exp − � � ∙ cos � ∙ 2� � , where � is the separation (in metric used in structural superposition is similar to the square root of MSE, but residues), � is the characteristic persistence length, and � is the period of uses the number of atoms in place of �. This is statistically justified only when oscillations. Optimally fitting parameters were � ≈ 33 and � ≈ 10 residues. atoms are entirely independent of each other, which is clearly not the case in Based on this, we chose to use a correlation length parameter for our RMSD general. -
View Full Page
The Journal of Neuroscience, August 1, 2001, 21(15):5813–5823 Expression of Protein Kinase C Inhibitor Blocks Cerebellar Long-Term Depression without Affecting Purkinje Cell Excitability in Alert Mice Jeroen Goossens,1,2 Herve´ Daniel,3 Armelle Rancillac,3 Johannes van der Steen,1 John Oberdick,4 Francis Cre´ pel,3 Christiaan I. De Zeeuw,5 and Maarten A. Frens1 1Department of Physiology, Neuroscience Institute, Erasmus University Rotterdam, 3000 DR Rotterdam, The Netherlands, 2Department of Medical Physics and Biophysics, University of Nijmegen, 6500 HB Nijmegen, The Netherlands, 3Laboratory of Neurobiology, Institute of Neuroscience, University of Paris IV, 75252 Paris, France, 4Department of Cell Biology, Ohio State University, Columbus, Ohio 43210, and 5Department of Anatomy, Neuroscience Institute, Erasmus University Rotterdam, 3000 DR Rotterdam, The Netherlands A longstanding but still controversial hypothesis is that long- innervation does not occur in cerebellar slices obtained from 3- term depression (LTD) of parallel fiber–Purkinje cell synapses in to 6-month-old mutants. These data indicate (1) that neither the cerebellum embodies part of the neuronal information stor- PKC inhibition nor the subsequent blockage of LTD induction age required for associative motor learning. Transgenic mice in disturbs the spontaneous discharge of Purkinje cells in alert which LTD is blocked by Purkinje cell-specific inhibition of mice, (2) that Purkinje cell-specific inhibition of PKC detains protein kinase C (PKC) (L7-PKCI mutants) do indeed show rather than prevents the developmental conversion from multi- impaired adaptation of their vestibulo-ocular reflex, whereas ple to mono-innervation of Purkinje cells by climbing fibers, and the dynamics of their eye movement performance are unaf- (3) that as a consequence the impaired motor learning as fected. -
Crystallization Communications Crystallization And
crystallization communications Acta Crystallographica Section F Structural Biology Crystallization and preliminary X-ray diffraction and Crystallization analysis of calexcitin from Loligo pealei: a neuronal Communications protein implicated in learning and memory ISSN 1744-3091 G. D. E. Beaven,a P. T. Erskine,a The neuronal protein calexcitin from the long-finned squid Loligo pealei has J. N. Wright,a F. Mohammed,a‡ been expressed in Escherichia coli and purified to homogeneity. Calexcitin is a R. Gill,a S. P. Wood,a J. Vernon,b 22 kDa calcium-binding protein that becomes up-regulated in invertebrates K. P. Gieseb and J. B. Coopera* following Pavlovian conditioning and is likely to be involved in signal transduction events associated with learning and memory. Recombinant squid aSchool of Biological Sciences, University of calexcitin has been crystallized using the hanging-drop vapour-diffusion Southampton, Bassett Crescent East, technique in the orthorhombic space group P212121. The unit-cell parameters Southampton SO16 7PX, England, and of a = 46.6, b = 69.2, c = 134.8 A˚ suggest that the crystals contain two monomers bWolfson Institute for Biomedical Research, per asymmetric unit and have a solvent content of 49%. This crystal form University College London, Cruciform Building, diffracts X-rays to at least 1.8 A˚ resolution and yields data of high quality using Gower Street, London WC1E 6BT, England synchrotron radiation. ‡ Present address: CRUK Institute for Cancer Studies, University of Birmingham, Vincent 1. Introduction Drive, Edgbaston, Birmingham B15 2TT, England. The protein calexcitin was originally identified in the photoreceptor neurons of the marine snail Hermissenda crassicornis as a 20 kDa protein that became up-regulated and phosphorylated following a Correspondence e-mail: Pavlovian training protocol (Nelson et al., 1990). -
NMR-Driven Secondary and Tertiary Structure Model of Ca -Loaded
BBRC Biochemical and Biophysical Research Communications 343 (2006) 520–524 www.elsevier.com/locate/ybbrc NMR-driven secondary and tertiary structure model of Ca2+-loaded calexcitin q Zoltan Gombos *, Kyoko L. Yap 1, Mitsuhiko Ikura, Avijit Chakrabartty Ontario Cancer Institute and Department of Medical Biophysics, University of Toronto, Ont., Canada M5G 1L7 Received 24 February 2006 Available online 10 March 2006 Abstract Calexcitin (CE) is a Ca2+-binding protein which is expressed in neuronal cells and is a member of the sarcoplasmic Ca2+-binding pro- tein subfamily. The peptide backbone of Ca2+-CE has been assigned by NMR and it shows that CE is composed of nine a-helices—form- ing four EF-hands and an additional helix near the C-terminus. A structural model of CE suggests the presence of a putative recessed hydrophobic pocket that may be involved in Ca2+-mediated protein–ligand interactions. This feature is unique to CE and is absent in other SCPs, such as those from Branchiostoma and Nereis, and from calerythrin. Ó 2006 Elsevier Inc. All rights reserved. Keywords: Calexcitin; Sarcoplasmic calcium-binding protein; Calcium; Backbone assignment; Structural model Calcium ions (Ca2+) are essential for the normal func- (termed CE) and those expressed in muscles (termed SCPs) tion of cells. Ca2+ signaling pathways often recruit special- [2]. This has also been supported by computational ized Ca2+-binding proteins (CaBPs), including Ca2+ studies [4]. sensors and buffers. Calexcitin (CE) is a cytosolic neuronal CE has been suggested to interact with at least two protein which may be implicated in learning, memory, and potential targets. -
Chapter 14 Phage Display Selection Of
M ETHODS IN MOLECULAR BIOLOGY™ Series Editor John M. Walker School of Life Sciences University of Hertfordshire Hat fi eld, Hertfordshire, AL10 9AB, UK For further volumes: http://www.springer.com/series/7651 Calcium-Binding Proteins and RAGE From Structural Basics to Clinical Applications Edited by Claus W. Heizmann Department of Pediatrics, Division of Clinical Chemistry and Biochemistry, University of Zürich, Zürich, Switzerland Editor Claus W. Heizmann Department of Pediatrics Division of Clinical Chemistry and Biochemistry University of Zürich Zürich, Switzerland ISSN 1064-3745 ISSN 1940-6029 (electronic) ISBN 978-1-62703-229-2 ISBN 978-1-62703-230-8 (eBook) DOI 10.1007/978-1-62703-230-8 Springer New York Heidelberg Dordrecht London Library of Congress Control Number: 2012952137 © Springer Science+Business Media New York 2013 This work is subject to copyright. All rights are reserved by the Publisher, whether the whole or part of the material is concerned, speci fi cally the rights of translation, reprinting, reuse of illustrations, recitation, broadcasting, reproduction on micro fi lms or in any other physical way, and transmission or information storage and retrieval, electronic adaptation, computer software, or by similar or dissimilar methodology now known or hereafter developed. Exempted from this legal reservation are brief excerpts in connection with reviews or scholarly analysis or material supplied specifi cally for the purpose of being entered and executed on a computer system, for exclusive use by the purchaser of the work. Duplication of this publication or parts thereof is permitted only under the provisions of the Copyright Law of the Publisher’s location, in its current version, and permission for use must always be obtained from Springer.