A Comparative Analysis of the Complete Chloroplast Genomes of Three Chrysanthemum Boreale Strains
Total Page:16
File Type:pdf, Size:1020Kb
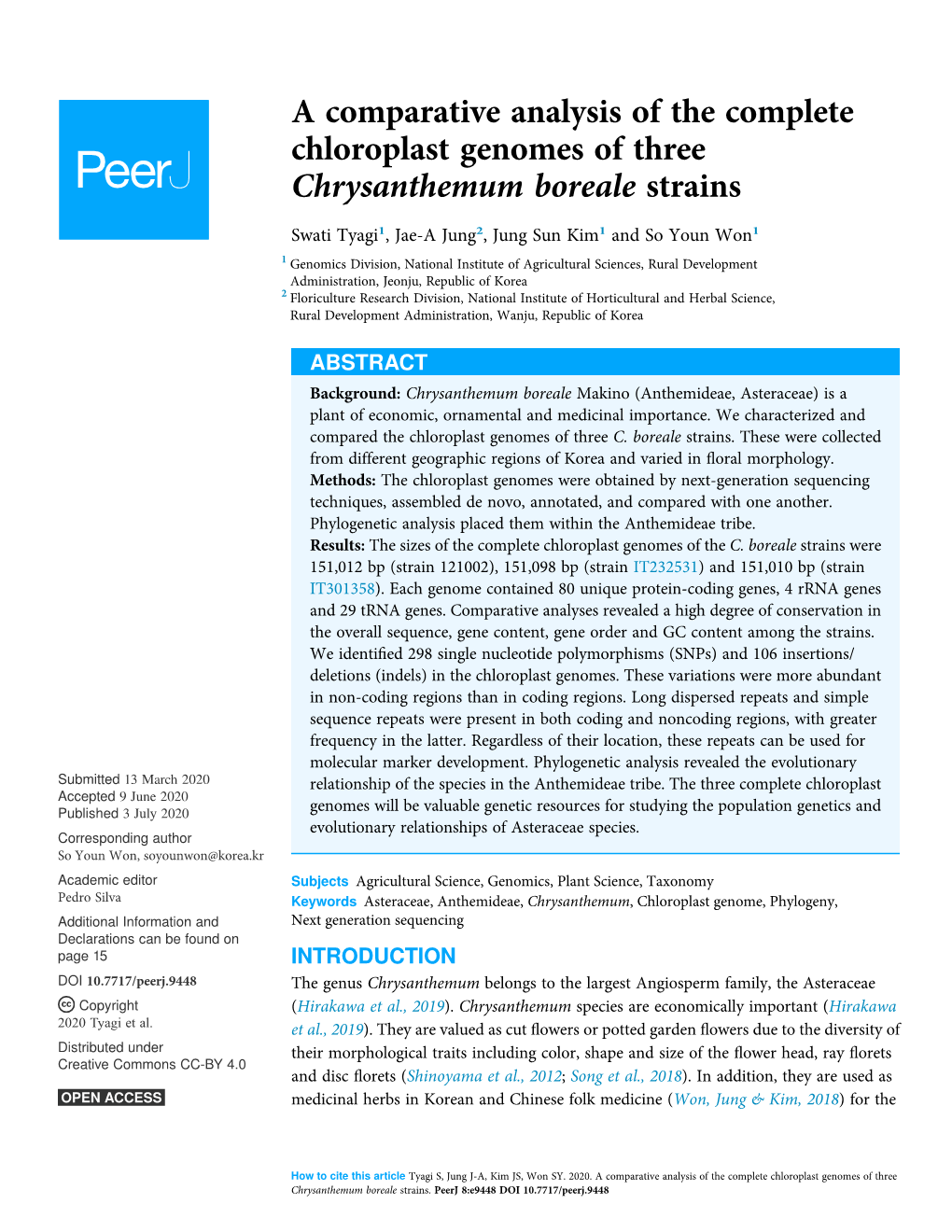
Load more
Recommended publications
-
Toronto Master Gardeners Ask Plant Id Questions
TORONTO MASTER GARDENERS ASK PLANT ID QUESTIONS Image Question Answer Growing in ditches beside a gravel road It is challenging to identify a plant from a single leaf, and I consulted our team in Township of Perry 25 minutes north of Master Gardeners, several of whom feel that the plant is likely some sort of Huntsville. Cant find it in any of our of dock. Consider the following: reference books. Leaves are emerging from ground singly and veins are deep ñ Rumex sanguineus var.sanguineus (red-veined or bloody red. dock). See the Missouri Botanical Garden monograph ñ Rumex obtusifolius (broadleaved dock/ bitter dock). See Illinois Wildflowers – Bitter Dock ñ Rumex aquaticus (Scottish dock). See Nature Gate’s Scottish Dock Another suggestion was this might be pokeweed (Phytolacca Americana). See Ohio State University’s Ohio Perennial and Biennial Weed May 2019 Guide – Common PokeweedClick on the above links and you'll see photos that show that these plants have leaves that resemble those of your mystery plant, in many respects. However, with docks and the common pokeweed, leaves generally emerge from the same clump, not singly. As well, these plants have lance-shaped leaves, which seem to differ quite a bit from the oblong-shaped leaf of shown in the photo you submitted.Finally, it is possible that the plant is related to dock, but is a sorrel (Rumex acetosa) - some sorrels have leaves that are shaped more like the leaf in your photo. For example, see Nature Gate's Common sorrel My neighbour gave me this plant, that I Your neighbour gave you a Bergenia cordifolia, commonly called Bergenia or planted las year. -
The Relation Between Road Crack Vegetation and Plant Biodiversity in Urban Landscape
Int. J. of GEOMATE, June, 2014, Vol. 6, No. 2 (Sl. No. 12), pp. 885-891 Geotech., Const. Mat. & Env., ISSN:2186-2982(P), 2186-2990(O), Japan THE RELATION BETWEEN ROAD CRACK VEGETATION AND PLANT BIODIVERSITY IN URBAN LANDSCAPE Taizo Uchida1, JunHuan Xue1,2, Daisuke Hayasaka3, Teruo Arase4, William T. Haller5 and Lyn A. Gettys5 1Faculty of Engineering, Kyushu Sangyo University, Japan; 2Suzhou Polytechnic Institute of Agriculture, China; 3Faculty of Agriculture, Kinki University, Japan; 4Faculty of Agriculture, Shinshu University, Japan; 5Center for Aquatic and Invasive Plants, University of Florida, USA ABSTRACT: The objective of this study is to collect basic information on vegetation in road crack, especially in curbside crack of road, for evaluating plant biodiversity in urban landscape. A curbside crack in this study was defined as a linear space (under 20 mm in width) between the asphalt pavement and curbstone. The species composition of plants invading curbside cracks was surveyed in 38 plots along the serial National Route, over a total length of 36.5 km, in Fukuoka City in southern Japan. In total, 113 species including native plants (83 species, 73.5%), perennial herbs (57 species, 50.4%) and woody plants (13 species, 11.5%) were recorded in curbside cracks. Buried seeds were also obtained from soil in curbside cracks, which means the cracks would possess a potential as seed bank. Incidentally, no significant differences were found in the vegetation characteristics of curbside cracks among land-use types (Kolmogorov-Smirnov Test, P > 0.05). From these results, curbside cracks would be likely to play an important role in offering habitat for plants in urban area. -
Artemisia Californica Less
I. SPECIES Artemisia californica Less. [Updated 2017] NRCS CODE: Subtribe: Artemisiinae ARCA11 Tribe: Anthemideae (FEIS CODE: Family: Asteraceae ARCAL) Order: Asterales Subclass: Asteridae Class: Magnoliopsida flowering heads spring growth seedling, March 2009 juvenile plant photos A. Montalvo flowering plant, November 2005 mature plant with flower buds August 2010 A. Subspecific taxa None. Artemisia californica Less. var. insularis (Rydb.) Munz is now recognized as Artemisia nesiotica P.H. Raven (Jepson eFlora 2017). B. Synonyms Artemisia abrotanoides Nuttall; A. fischeriana Besser; A. foliosa Nuttall; Crossostephium californicum (Lessing) Rydberg (FNA 2017). C. Common name California sagebrush. The common name refers to its strong, sage-like aroma and endemism to California and Baja California. Other names include: coastal sage, coast sage, coast sagebrush (Painter 2016). D. Taxonomic relationships The FNA (2017) places this species in subgenus Artemisia . The molecular phylogeny of the genus has improved the understanding of relationships among the many species of Artemisia and has, at times, placed the species in subgenus Tridentadae; morphology of the inflorescences and flowers alone does not place this species with its closest relatives (Watson et al. 2002). The detailed phylogeny is not completely resolved (Hayat et al. 2009). E. Related taxa in region There are 18 species and a total of 31 taxa (including infrataxa) of Artemisia in southern California, all of which differ clearly from A. californica in habitat affinity, structure, or both (Munz 1974, Jepson eFlora 2017). Within subgenus Artemisia (as per FNA 2017), A. nesiotica from the Channel Islands is the most similar and was once considered part of A. californica ; it can be distinguished by its wider leaves with flat leaf margins (not rolled under). -
Achillea Millefolium L
SPECIES Achillea millefolium L. Tribe: Anthemideae Family: Asteraceae USDA CODE: Order: Asterales Subclass: Asteridae ACMI2 Class: Magnoliopsida FEIS CODE: D. Kopp 2009 San Bernardino Mtns. ACHMIL A. Montalvo 2010 Monterey Co. coast; tripinnate, pubescent form A. Montalvo 2010 Monterey Co. Subspecific taxa JepsonOnline 2010 and FNA 2010 do not recognize subspecific taxa of A. millefolium . The USDA PLANTS database (viewed Sept. 24, 2010) recognizes 12 subspecific taxa as occurring in North America: Taxon introduced and naturalized in North America (thought to be native to Europe): NRCS CODES: 1. A. m. L. var. millefolium 1. ACMIM2 Taxa native to California: 2. ACMIA 2. A. m. L. var. alpicola (Rydb.) Garrolt 3. ACMIA2 3. A. m. L. var. arenicola (Heller) Nobs 4. ACMIC 4. A. m. L. var. californica (Pollard) Jepson 5. ACMIG 5. A. m. L. var. gigantea (Pollard) Nobs 6. ACMIO 6. A. m. L. var. occidentalis (DC.) Hyl. 7. ACMIP 7. A. m. L. var. pacifica (Rydb.) G.N.Jones 8. ACMIP2 8. A. m. L. var. puberula (Rydb.) Nobs. 9. ACMIB Additional taxa outside California (mostly northerly): 10. ACMIL2 9. A. m. L. var. borealis (Bong.) Farw. 11. ACMIM5 10. A. m. L. var. litoralis (Ehrend.) Nobs 12. ACMIN 11. A. m. L. var. megacephala (Raup) Bolvin. 12. A. m. L. var. nigrescens E. Mey. Synonyms (USDA PLANTS) 2. A. alpicola (Rydb.) Rydb.; A. fusca Rydb.; A. lanulosa Nutt. ssp. alpicola (Rydb.) D.D. Keck; A. l. Nutt. var. alpicola Rydb.; A. m. L. var. fusca (Rydb.) G.N. Jones; A. subalpina Greene Taxa numbered as above 3. -
Patterns of Resource Allocation in Different Habitats of Kalimeris Intergrifolia in Northeast China Z
Instituto Nacional de Investigación y Tecnología Agraria y Alimentaria (INIA) Spanish Journal of Agricultural Research 2011 9(4), 1224-1232 Available online at www.inia.es/sjar ISSN: 1695-971-X doi: http://dx.doi.org/10.5424/sjar/20110904-162-11 eISSN: 2171-9292 Patterns of resource allocation in different habitats of Kalimeris intergrifolia in Northeast China Z. N. Yuan1,2, J. M. Lu1*, J. Y. Chen1 and S. Z. Jiang2 1College of Life Sciences, Northeast Normal University. Changchun 130024. P. R. China 2 Key Laboratory of Molecular Cytogenetics and Genetic Breeding of Heilongjiang Province; College of life Sciences and Technology, Harbin Normal University. Harbin 150025. P. R. China Abstract Understanding parameters that drive plant resource allocation for reproduction in potentially economically and environ- mentally important species, such as Kalimeris intergrifolia, is essential to maximize production rates. Hence, this study evaluates the characteristics in reproductive resource allocation of two K. intergrifolia communities at a saline-alkali open meadow and at a semi-enclosed secondary broad-leaved forest fringe, in the Songnen plains region of northeast China. Ramets from each habitat type were sampled at three intervals during the ripening stage (June-October). Relative resource distribution was quantified by measuring the dry weight of the above-ground ramet components, including the stem, leaf, corymb and seeds. The results indicated high variability in the distribution of resource allocation for both types, with larger phenotypic plasticity being recorded for the forest fringe than the open meadow. However, the allocation of re- sources into reproductive organs was higher in the open meadow than in the forest fringe, demonstrating that the open community was advantageous to the reproduction. -
Chapter 4 Phytogeography of Northeast Asia
Chapter 4 Phytogeography of Northeast Asia Hong QIAN 1, Pavel KRESTOV 2, Pei-Yun FU 3, Qing-Li WANG 3, Jong-Suk SONG 4 and Christine CHOURMOUZIS 5 1 Research and Collections Center, Illinois State Museum, 1011 East Ash Street, Springfield, IL 62703, USA, e-mail: [email protected]; 2 Institute of Biology and Soil Science, Russian Academy of Sciences, Vladivostok, 690022, Russia, e-mail: [email protected]; 3 Institute of Applied Ecology, Chinese Academy of Sciences, P.O. Box 417, Shenyang 110015, China; 4 Department of Biological Science, College of Natural Sciences, Andong National University, Andong 760-749, Korea, e-mail: [email protected]; 5 Department of Forest Sciences, University of British Columbia, 3041-2424 mail Mall, Vancouver, B.C., V6T 1Z4, Canada, e-mail: [email protected] Abstract: Northeast Asia as defined in this study includes the Russian Far East, Northeast China, the northern part of the Korean Peninsula, and Hokkaido Island (Japan). We determined the species richness of Northeast Asia at various spatial scales, analyzed the floristic relationships among geographic regions within Northeast Asia, and compared the flora of Northeast Asia with surrounding floras. The flora of Northeast Asia consists of 971 genera and 4953 species of native vascular plants. Based on their worldwide distributions, the 971 gen- era were grouped into fourteen phytogeographic elements. Over 900 species of vascular plants are endemic to Northeast Asia. Northeast Asia shares 39% of its species with eastern Siberia-Mongolia, 24% with Europe, 16.2% with western North America, and 12.4% with eastern North America. -
Sequencing and Analysis of Chrysanthemum Carinatum Schousb and Kalimeris Indica
molecules Article Sequencing and Analysis of Chrysanthemum carinatum Schousb and Kalimeris indica. The Complete Chloroplast Genomes Reveal Two Inversions and rbcL as Barcoding of the Vegetable Xia Liu * ID , Boyang Zhou, Hongyuan Yang, Yuan Li, Qian Yang, Yuzhuo Lu and Yu Gao State Key Laboratory of Food Nutrition and Safety, Key Laboratory of Food Nutrition and Safety, Ministry of Education of China, College of Food Engineering and Biotechnology, Tianjin University of Science &Technology, Tianjin 300457, China; [email protected] (B.Z.); [email protected] (H.Y.); [email protected] (Y.L.); [email protected] (Q.Y.); [email protected] (Y.L.); [email protected] (Y.G.) * Correspondence: [email protected]; Tel.: +86-022-6091-2406 Received: 20 April 2018; Accepted: 31 May 2018; Published: 5 June 2018 Abstract: Chrysanthemum carinatum Schousb and Kalimeris indica are widely distributed edible vegetables and the sources of the Chinese medicine Asteraceae. The complete chloroplast (cp) genome of Asteraceae usually occurs in the inversions of two regions. Hence, the cp genome sequences and structures of Asteraceae species are crucial for the cp genome genetic diversity and evolutionary studies. Hence, in this paper, we have sequenced and analyzed for the first time the cp genome size of C. carinatum Schousb and K. indica, which are 149,752 bp and 152,885 bp, with a pair of inverted repeats (IRs) (24,523 bp and 25,003) separated by a large single copy (LSC) region (82,290 bp and 84,610) and a small single copy (SSC) region (18,416 bp and 18,269), respectively. In total, 79 protein-coding genes, 30 distinct transfer RNA (tRNA) genes, four distinct rRNA genes and two pseudogenes were found not only in C. -
Paper Version of Palos Verdes
Selected Plants Native to Palos Verdes Peninsula (C.M. Rodrigue, 07/26/11) http://www.csulb.edu/geography/PV/ Succulents (plants with fleshy, often liquid-saturated leaves and/or stems. These features can be found in a variety of life forms, including annual herbaceous plants, vines, shrubs, and trees, as well as cacti) Herbaceous plants (non-woody, though there may be a woody caudex or basal stem and root -- annual growth dies back each year, resprouting in perennial or biennial plants, or the plant dies and is replaced by a new generation each year in the case of annual plants) Extremely tiny plant. Stems only about 2-6 cm tall, occasionally as much as 10 cm, leaves only 1-3 mm long (can get up to 6 mm long), fleshy, found at the plant's base or on the stems, shape generally ovate (egg-shaped), may have a blunt rounded end or a fine acute tip. The leaves are arranged oppositely, not alternately. The plant is green when new but ages to red or pink. Tiny flower (0.5- 2 mm) borne in leaf axils, usually just one per leaf pair on a pedicel (floral stem) less than 6 mm long. Two or 3 petals and 3 or 4 sepals. Flowers February to May. Annual herb. Found in open areas, in rocky nooks and crannies, and sometimes in vernal ponds (temporary pools that form after a rain and then slowly evaporate). Crassula connata (Crassulaceae): pygmy stonecrop or pygmy-weed or sand pygmyweed Leaves converted into scales along stems, which are arranged alternately and overlap. -
Molecular Phylogeny of Subtribe Artemisiinae (Asteraceae), Including Artemisia and Its Allied and Segregate Genera Linda E
University of Nebraska - Lincoln DigitalCommons@University of Nebraska - Lincoln Faculty Publications in the Biological Sciences Papers in the Biological Sciences 9-26-2002 Molecular phylogeny of Subtribe Artemisiinae (Asteraceae), including Artemisia and its allied and segregate genera Linda E. Watson Miami University, [email protected] Paul E. Bates University of Nebraska-Lincoln, [email protected] Timonthy M. Evans Hope College, [email protected] Matthew M. Unwin Miami University, [email protected] James R. Estes University of Nebraska State Museum, [email protected] Follow this and additional works at: http://digitalcommons.unl.edu/bioscifacpub Watson, Linda E.; Bates, Paul E.; Evans, Timonthy M.; Unwin, Matthew M.; and Estes, James R., "Molecular phylogeny of Subtribe Artemisiinae (Asteraceae), including Artemisia and its allied and segregate genera" (2002). Faculty Publications in the Biological Sciences. 378. http://digitalcommons.unl.edu/bioscifacpub/378 This Article is brought to you for free and open access by the Papers in the Biological Sciences at DigitalCommons@University of Nebraska - Lincoln. It has been accepted for inclusion in Faculty Publications in the Biological Sciences by an authorized administrator of DigitalCommons@University of Nebraska - Lincoln. BMC Evolutionary Biology BioMed Central Research2 BMC2002, Evolutionary article Biology x Open Access Molecular phylogeny of Subtribe Artemisiinae (Asteraceae), including Artemisia and its allied and segregate genera Linda E Watson*1, Paul L Bates2, Timothy M Evans3, -
Astereae, Asteraceae) Using Molecular Phylogeny of ITS
Turkish Journal of Botany Turk J Bot (2015) 39: 808-824 http://journals.tubitak.gov.tr/botany/ © TÜBİTAK Research Article doi:10.3906/bot-1410-12 Relationships and generic delimitation of Eurasian genera of the subtribe Asterinae (Astereae, Asteraceae) using molecular phylogeny of ITS 1, 2,3 4 Elena KOROLYUK *, Alexey MAKUNIN , Tatiana MATVEEVA 1 Central Siberian Botanical Garden, Siberian Branch of Russian Academy of Sciences, Novosibirsk, Russia 2 Institute of Molecular and Cell Biology, Siberian Branch of Russian Academy of Sciences, Novosibirsk, Russia 3 Theodosius Dobzhansky Center for Genome Bioinformatics, Saint Petersburg State University, Saint Petersburg, Russia 4 Department of Genetics & Biotechnology, Saint Petersburg State University, Saint Petersburg, Russia Received: 12.10.2014 Accepted/Published Online: 02.04.2015 Printed: 30.09.2015 Abstract: The subtribe Asterinae (Astereae, Asteraceae) includes highly variable, often polyploid species. Recent findings based on molecular methods led to revision of its volume. However, most of these studies lacked species from Eurasia, where a lot of previous taxonomic treatments of the subtribe exist. In this study we used molecular phylogenetics methods with internal transcribed spacer (ITS) as a marker to resolve evolutionary relations between representatives of the subtribe Asterinae from Siberia, Kazakhstan, and the European part of Russia. Our reconstruction revealed that a clade including all Asterinae species is paraphyletic. Inside this clade, there are species with unresolved basal positions, for example Erigeron flaccidus and its relatives. Moreover, several well-supported groups exist: group of the genera Galatella, Crinitaria, Linosyris, and Tripolium; group of species of North American origin; and three related groups of Eurasian species: typical Eurasian asters, Heteropappus group (genera Heteropappus, Kalimeris), and Asterothamnus group (genera Asterothamnus, Rhinactinidia). -
A Legacy of Plants N His Short Life, Douglas Created a Tremendous Legacy in the Plants That He Intro (P Coulteri) Pines
The American lIorHcullural Sociely inviles you Io Celehrate tbe American Gardener al our 1999 Annual Conference Roston" Massachusetts June 9 - June 12~ 1999 Celebrate Ute accompHsbenls of American gardeners in Ute hlsloric "Cay Upon lhe 1Iill." Join wah avid gardeners from. across Ute counlrg lo learn new ideas for gardening excellence. Attend informa-Hve ledures and demonslraHons by naHonally-known garden experts. Tour lhe greal public and privale gardens in and around Roslon, including Ute Arnold Arborelum and Garden in Ute Woods. Meet lhe winners of AIlS's 1999 naHonJ awards for excellence in horHcullure. @ tor more informaHon, call1he conference regislrar al (800) 777-7931 ext 10. co n t e n t s Volume 78, Number 1 • '.I " Commentary 4 Hellebores 22 Members' Forum 5 by C. Colston Burrell Staghorn fern) ethical plant collecting) orchids. These early-blooming pennnials are riding the crest of a wave ofpopularity) and hybridizers are News from AHS 7 busy working to meet the demand. Oklahoma Horticultural Society) Richard Lighty) Robert E. Lyons) Grecian foxglove. David Douglas 30 by Susan Davis Price Focus 9 Many familiar plants in cultivation today New plants for 1999. are improved selections of North American species Offshoots 14 found by this 19th-century Scottish expLorer. Waiting for spring in Vermont. Bold Plants 37 Gardeners Information Service 15 by Pam Baggett Houseplants) transplanting a ginkgo tree) Incorporating a few plants with height) imposing starting trees from seed) propagating grape vines. foliage) or striking blossoms can make a dramatic difference in any landscape design. Mail-Order Explorer 16 Heirloom flowers and vegetables. -
Achene Micro-Morphology of Anthemis (Asteraceae) and Its Allies in Iran with Emphasis on Systematics
INTERNATIONAL JOURNAL OF AGRICULTURE & BIOLOGY 1560–8530/2007/09–3–486–488 http://www.fspublishers.org Achene Micro-morphology of Anthemis (Asteraceae) and its Allies in Iran with Emphasis on Systematics ABDOLKARIM CHEHREGANI1 AND NATEGHEH MAHANFAR Laboratory of Plant, Cell and Molecular Biology, Department of Biology, Faculty of Sciences, Bu-Ali University, Hamedan, Iran 1Correspondence author’s e-mail: [email protected] ABSTRACT Anthemideae is a large tribe of Astraceae and poses difficulties in recognizing and classifying its members based on morphological characters. This research was focused on evaluating achene peculiarities for systematic purpose. Achene micro- morphological characteristics such as size, shape, papuse, sulcuse, lacune, etc., were considered useful in species recognition. Lacunas characters in each studied taxa were specific and regarded as good separator character in the studied taxa of this tribe. Based on data, Tanacetum kotschyi was considered as a problematic species that needs to be studied further. Key Words: Anthemideae; Anthemis; SEM; Achene; Systematic INTRODUCTION were deposited in Tehran University Herbarium and Bu-Ali Sina University (Table I). The achenes were coated with a Anthemideae is the seventh largest tribe of Asteraceae thin layer of gold-paladium and studied with a JEOL-840 with about 109 genera and 1740 species worldwide Scanning Electron Microscope (Japan) at Sanati Sharif (Bremer, 1994; Tahir et al., 2002). The tribe and shows a University, Tehran, Iran. Achene morphological and micro- temperate distribution (Heywood & Humphries, 1977; morphological characters such as size, shape, papuse, Bremer & Humphries, 1993; Francisco-Ortega et al., 2001). sulcuse, lacune and etc., were compared with prepared The members of this tribe have been studied by several micrograph.