Magnetofossil Taphonomy with Ferromagnetic Resonance Spectroscopy ⁎ Robert E
Total Page:16
File Type:pdf, Size:1020Kb
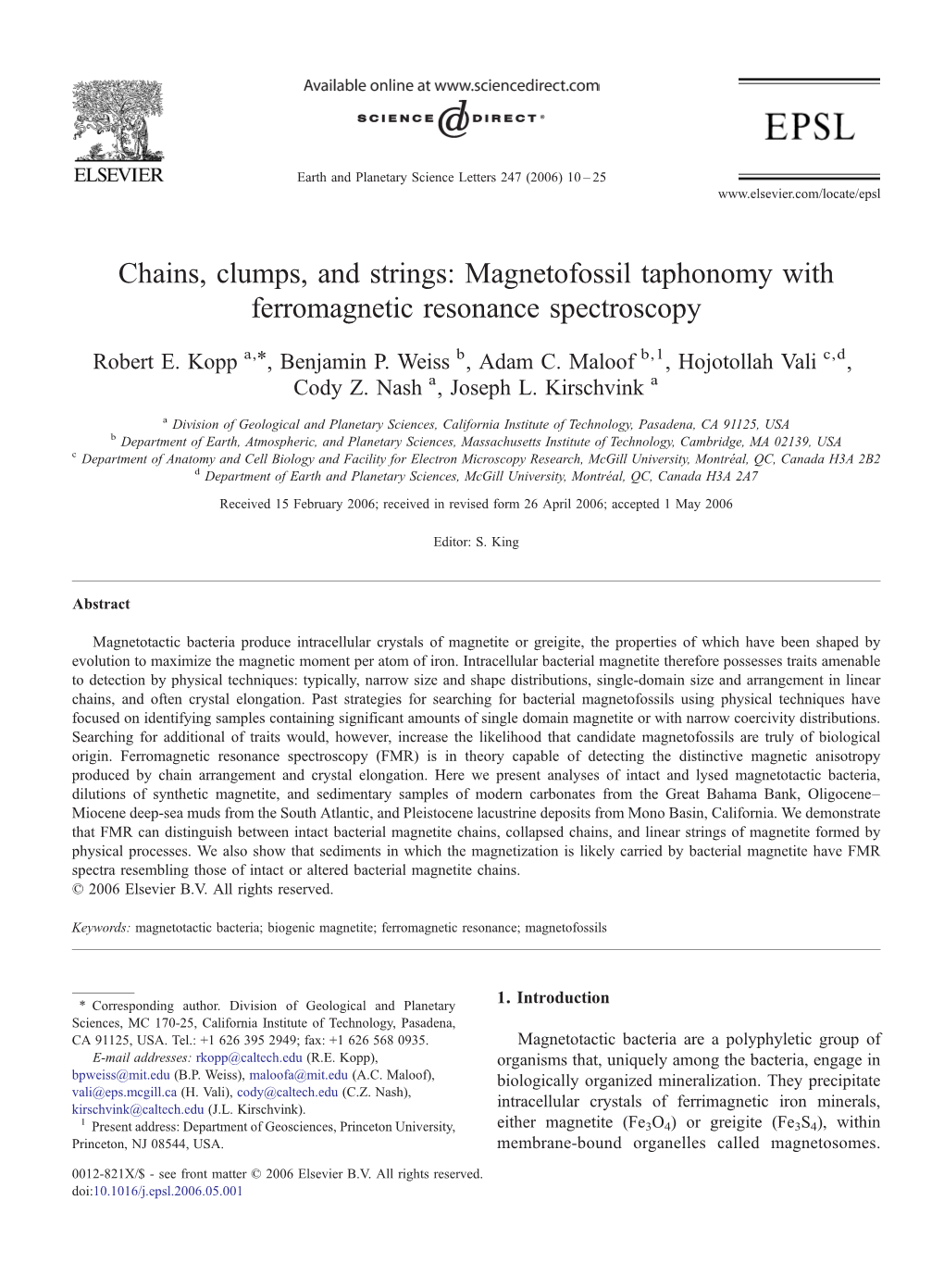
Load more
Recommended publications
-
361: Crystallography and Diffraction
361: Crystallography and Diffraction Michael Bedzyk Department of Materials Science and Engineering Northwestern University February 2, 2021 Contents 1 Catalog Description3 2 Course Outcomes3 3 361: Crystallography and Diffraction3 4 Symmetry of Crystals4 4.1 Types of Symmetry.......................... 4 4.2 Projections of Symmetry Elements and Point Groups...... 9 4.3 Translational Symmetry ....................... 17 5 Crystal Lattices 18 5.1 Indexing within a crystal lattice................... 18 5.2 Lattices................................. 22 6 Stereographic Projections 31 6.1 Projection plane............................ 33 6.2 Point of projection........................... 33 6.3 Representing Atomic Planes with Vectors............. 36 6.4 Concept of Reciprocal Lattice .................... 38 6.5 Reciprocal Lattice Vector....................... 41 7 Representative Crystal Structures 48 7.1 Crystal Structure Examples ..................... 48 7.2 The hexagonal close packed structure, unlike the examples in Figures 7.1 and 7.2, has two atoms per lattice point. These two atoms are considered to be the motif, or repeating object within the HCP lattice. ............................ 49 1 7.3 Voids in FCC.............................. 54 7.4 Atom Sizes and Coordination.................... 54 8 Introduction to Diffraction 57 8.1 X-ray .................................. 57 8.2 Interference .............................. 57 8.3 X-ray Diffraction History ...................... 59 8.4 How does X-ray diffraction work? ................. 59 8.5 Absent -
Magnetotactic Bacteria and Their Application in Medicine
Chem cal ist si ry y & h P B f i o o Dasdag and Bektas. J Phys Chem Biophys 2014, 4:2 p l h a Journal of Physical Chemistry & y n s r DOI: 10.4172/2161-0398.1000141 i u c o s J ISSN: 2161-0398 Biophysics ResearchReview Article Article OpenOpen Access Access Magnetotactic Bacteria and their Application in Medicine Suleyman Dasdag1* and Hava Bektas2 1Department of Biophysics, Medical School of Dicle University, Diyarbakir, Turkey 2Department of Biophysics, Medical School of Yuzuncu Yil University, Van / Turkey Abstract It is a known fact how the magnetic field of the Earth is very important for life. Relation between living systems and the earth magnetic field has been investigated for many years. Birds and their migration routes are the first one of the things that comes to mind when we state living things. The Earth’s magnetic field is still accepted to be the main factor for birds and other flying living beings to complete their travels correctly. The changes in migration routes, which are observed from time to time, are sometimes said to be due to the changes in the magnetic field. However, no light has been shed to this matter yet. The Earth’s magnetic field has not been sufficiently studied, and its role on small living models such as bacteria has not been adequately discussed. One of the best examples in this field is relation between the Earth’s magnetic field and “magnetotactic bacteria (MTB)”, which were discovered by Salvatore Bellini in 1963. Currently, it is claimed that magnetotactic bacteria have a widespread use in microbiology, mineralogy, limnology, physics, biophysics, chemistry, biochemistry, geology, crystallography, and astrobiology. -
Life with Compass: Diversity and Biogeography of Magnetotactic Bacteria
bs_bs_banner Environmental Microbiology (2014) 16(9), 2646–2658 doi:10.1111/1462-2920.12313 Minireview Life with compass: diversity and biogeography of magnetotactic bacteria Wei Lin,1,2 Dennis A. Bazylinski,3 Tian Xiao,2,4 the present-day biogeography of MTB, and the ruling Long-Fei Wu2,5 and Yongxin Pan1,2* parameters of their spatial distribution, will eventu- 1Biogeomagnetism Group, Paleomagnetism and ally help us predict MTB community shifts with envi- Geochronology Laboratory, Key Laboratory of the ronmental changes and assess their roles in global Earth’s Deep Interior, Institute of Geology and iron cycling. Geophysics, Chinese Academy of Sciences, Beijing 100029, China. 2France-China Bio-Mineralization and Nano-Structures Introduction Laboratory, Chinese Academy of Sciences, Beijing Iron is the fourth most common element in the Earth’s 100029, China. crust and a crucial nutrient for almost all known organ- 3 School of Life Sciences, University of Nevada at Las isms. The cycling of iron is one of the key processes in the Vegas, Las Vegas, NV, USA. Earth’s biogeochemical cycles. A number of organisms 4 Key Laboratory of Marine Ecology & Environmental synthesize iron minerals and play essential roles in global Sciences, Institute of Oceanology, Chinese Academy of iron cycling (Westbroek and de Jong, 1983; Winklhofer, Sciences, Qingdao, China. 2010). One of the most interesting examples of these 5 Laboratoire de Chimie Bactérienne, Aix-Marseille types of organisms are the magnetotactic bacteria (MTB), Université, CNRS, Marseille Cedex, France. a polyphyletic group of prokaryotes that are ubiquitous in aquatic and sedimentary environments (Bazylinski Summary and Frankel, 2004; Bazylinski et al., 2013). -
Investigation of the Magnetosome Biomineralization in Magnetotactic Bacteria Using Graphene Liquid Cell – Transmission Electron Microscopy
Nanoscale Investigation of the Magnetosome Biomineralization in Magnetotactic Bacteria using Graphene Liquid Cell – Transmission Electron Microscopy Journal: Nanoscale Manuscript ID NR-ART-10-2018-008647.R1 Article Type: Paper Date Submitted by the 27-Nov-2018 Author: Complete List of Authors: Firlar, Emre; University of Illinois at Chicago, Department of Bioengineering; University of Illinois at Chicago, Department of Mechanical and Industrial Engineering Ouy, Meagan; University of Illinois at Chicago, Department of Bioengineering Bogdanowicz, Agata; University of Illinois at Chicago, Department of Bioengineering Covnot, Leigha; University of Illinois at Chicago, Department of Bioengineering Song, Boao; University of Illinois at Chicago, Mechanical Engineering Department Nadkarni, Yash; University of Illinois at Chicago, Department of Bioengineering Shahbazian-Yassar, Reza; University of Illinois at Chicago, Mechanical and Industrial Engineering; University of Illinois at Chicago Shokuhfar, Tolou; University of Illinois at Chicago, Department of Bioengineering; Michigan Technological University, Department of Mechanical Engineering Page 1 of 8 Please doNanoscale not adjust margins Journal Name ARTICLE Investigation of the Magnetosome Biomineralization in Magnetotactic Bacteria using Graphene Liquid Cell – Transmission Electron Microscopy Received 00th January 20xx, Accepted 00th January 20xx Emre Firlara,b*, Meagan Ouya, Agata Bogdanowicza, Leigha Covnota, Boao Songb, Yash Nadkarnia, DOI: 10.1039/x0xx00000x Reza Shahbazian-Yassarb*, -
Additive Effects of Alkali Metals on Cu-Modified Ch3nh3pbi3−Δclδ
RSC Advances PAPER View Article Online View Journal | View Issue Additive effects of alkali metals on Cu-modified CH3NH3PbI3ÀdCld photovoltaic devices† Cite this: RSC Adv.,2019,9,24231 Naoki Ueoka, Takeo Oku * and Atsushi Suzuki We investigated the addition of alkali metal elements (namely Na+,K+,Rb+, and Cs+) to Cu-modified CH3NH3PbI3ÀdCld photovoltaic devices and their effects on the photovoltaic properties and electronic structure. The open-circuit voltage was increased by CuBr2 addition to the CH3NH3PbI3ÀdCld precursor solution. The series resistance was decreased by simultaneous addition of CuBr2 and RbI, which increased the external quantum efficiencies in the range of 300–500 nm, and the short-circuit current density. The energy gap of the perovskite crystal increased through CuBr2 addition, which we also confirmed by first-principles calculations. Charge carrier generation was observed in the range of 300– Received 25th April 2019 500 nm as an increase of the external quantum efficiency, owing to the partial density of states Accepted 24th July 2019 contributed by alkali metal elements. Calculations suggested that the Gibbs energies were decreased by DOI: 10.1039/c9ra03068a incorporation of alkali metal elements into the perovskite crystals. The conversion efficiency was Creative Commons Attribution 3.0 Unported Licence. rsc.li/rsc-advances maintained for 7 weeks for devices with added CuBr2 and RbI. PbI2 +CH3NH3I + 2CH3NH3Cl / CH3NH3PbI3 Introduction + 2CH3NH3Cl (g)[ (140 C)(2) Studies of methylammonium lead halide perovskite solar x y / cells started in 2009, when a conversion efficiency of 3.9% PbI2 + CH3NH3I+ CH3NH3Cl (CH3NH3)x+yPbI2+xCly 1 / [ was reported. Some devices have since yielded conversion CH3NH3PbI3 +CH3NH3Cl (g) (140 C) (3) efficiencies of more than 20% as studies have expanded This article is licensed under a 2–5 Owing to the formation of intermediates and solvent evap- globally, with expectations for these perovskite solar cells 6–9 oration, perovskite grains are gradually formed by annealing. -
Pharmaceutico Analytical Study of Udayabhaskara Rasa
INTERNATIONAL AYURVEDIC MEDICAL JOURNAL Research Article ISSN: 2320 5091 Impact Factor: 4.018 PHARMACEUTICO ANALYTICAL STUDY OF UDAYABHASKARA RASA Gopi Krishna Maddikera1, Ranjith. B. M2, Lavanya. S. A3, Parikshitha Navada4 1Professor & HOD, Dept of Rasashastra & Bhaishajya Kalpana, S.J.G Ayurvedic Medical College & P.G Centre, Koppal, Karnataka, India 2Ayurveda Vaidya; 3Ayurveda Vaidya; 43rd year P.G Scholar, T.G.A.M.C, Bellary, Karnataka, India Email: [email protected] Published online: January, 2019 © International Ayurvedic Medical Journal, India 2019 ABSTRACT Udayabhaskara rasa is an eccentric formulation which is favourable in the management of Amavata. Regardless of its betterment in the management of Amavata, no research work has been carried out till date. The main aim of this study was preparation of Udayabhaskara Rasa as disclosed in the classics & Physico-chemical analysis of Udayabhaskara Rasa. Udayabhaskara Rasa was processed using Kajjali, Vyosha, dwikshara, pancha lavana, jayapala and beejapoora swarasa. The above ingredients were mixed to get a homogenous mixture of Udayab- haskara Rasa which was given 1 Bhavana with beejapoora swarasa and later it is dried and stored in air-tight container. The Physico chemical analysis of Udayabhaskara Rasa before (UB-BB) and after bhavana (UB-AB) was done. Keywords: Udayabhaskara Rasa, XRD, FTIR, SEM-EDAX. INTRODUCTION Rasashastra is a branch of Ayurveda which deals with Udayabhaskara Rasa. In the present study the formu- metallo-mineral preparations aimed at achieving Lo- lation is taken from the text brihat nighantu ratna- havada & Dehavada. These preparations became ac- kara. The analytical study reveals the chemical com- ceptable due to its assimilatory property in the minute position of the formulations as well as their concentra- doses. -
Magnetic Properties of Uncultivated Magnetotactic Bacteria and Their Contribution to a Stratified Estuary Iron Cycle
ARTICLE Received 6 Feb 2014 | Accepted 25 Jul 2014 | Published 1 Sep 2014 DOI: 10.1038/ncomms5797 Magnetic properties of uncultivated magnetotactic bacteria and their contribution to a stratified estuary iron cycle A.P. Chen1, V.M. Berounsky2, M.K. Chan3, M.G. Blackford4, C. Cady5,w, B.M. Moskowitz6, P. Kraal7, E.A. Lima8, R.E. Kopp9, G.R. Lumpkin4, B.P. Weiss8, P. Hesse1 & N.G.F. Vella10 Of the two nanocrystal (magnetosome) compositions biosynthesized by magnetotactic bacteria (MTB), the magnetic properties of magnetite magnetosomes have been extensively studied using widely available cultures, while those of greigite magnetosomes remain poorly known. Here we have collected uncultivated magnetite- and greigite-producing MTB to determine their magnetic coercivity distribution and ferromagnetic resonance (FMR) spectra and to assess the MTB-associated iron flux. We find that compared with magnetite-producing MTB cultures, FMR spectra of uncultivated MTB are characterized by a wider empirical parameter range, thus complicating the use of FMR for fossilized magnetosome (magnetofossil) detection. Furthermore, in stark contrast to putative Neogene greigite magnetofossil records, the coercivity distributions for greigite-producing MTB are fundamentally left-skewed with a lower median. Lastly, a comparison between the MTB-associated iron flux in the investigated estuary and the pyritic-Fe flux in the Black Sea suggests MTB play an important, but heretofore overlooked role in euxinic marine system iron cycle. 1 Department of Environment and Geography, Macquarie University, North Ryde, New South Wales 2109, Australia. 2 Graduate School of Oceanography, University of Rhode Island, Narragansett, Rhode Island 02882, USA. 3 School of Physics and Astronomy, University of Minnesota, Minneapolis, Minnesota 55455, USA. -
A Perspective on Underlying Crystal Growth Mechanisms in Biomineralization: Solution Mediated Growth Versus Nanosphere Particle Accretion
CrystEngComm A perspective on underlying crystal growth mechanisms in biomineralization: solution mediated growth versus nanosphere particle accretion Journal: CrystEngComm Manuscript ID: CE-HIG-07-2014-001474.R1 Article Type: Highlight Date Submitted by the Author: 01-Dec-2014 Complete List of Authors: Gal, Assaf; Weizmann Institute of Science, Structural Biology Weiner, Steve; Weizmann Institute of Science, Structural Biology Addadi, Lia; Weizmann Institute of Science, Structural Biology Page 1 of 23 CrystEngComm A perspective on underlying crystal growth mechanisms in biomineralization: solution mediated growth versus nanosphere particle accretion Assaf Gal, Steve Weiner, and Lia Addadi Department of Structural Biology, Weizmann Institute of Science, Rehovot, Israel 76100 Abstract Many organisms form crystals from transient amorphous precursor phases. In the cases where the precursor phases were imaged, they consist of nanosphere particles. Interestingly, some mature biogenic crystals also have nanosphere particle morphology, but some are characterized by crystallographic faces that are smooth at the nanometer level. There are also biogenic crystals that have both crystallographic faces and nanosphere particle morphology. This highlight presents a working hypothesis, stating that some biomineralization processes involve growth by nanosphere particle accretion, where amorphous nanoparticles are incorporated as such into growing crystals and preserve their morphology upon crystallization. This process produces biogenic crystals with a nanosphere particle morphology. Other biomineralization processes proceed by ion-by-ion growth, and some cases of biological crystal growth involve both processes. We also identify several biomineralization processes which do not seem to fit this working hypothesis. It is our hope that this highlight will inspire studies that will shed more light on the underlying crystallization mechanisms in biology. -
An Atomistic Study of Phase Transition in Cubic Diamond Si Single Crystal T Subjected to Static Compression ⁎ Dipak Prasad, Nilanjan Mitra
Computational Materials Science 156 (2019) 232–240 Contents lists available at ScienceDirect Computational Materials Science journal homepage: www.elsevier.com/locate/commatsci An atomistic study of phase transition in cubic diamond Si single crystal T subjected to static compression ⁎ Dipak Prasad, Nilanjan Mitra Indian Institute of Technology Kharagpur, Kharagpur 721302, India ARTICLE INFO ABSTRACT Keywords: It is been widely experimentally reported that Si under static compression (typically in a Diamond Anvil Setup- Molecular dynamics DAC) undergoes different phase transitions. Even though numerous interatomic potentials are used fornu- Phase transition merical studies of Si under different loading conditions, the efficacy of different available interatomic potentials Hydrostatic and Uniaxial compressive loading in determining the phase transition behavior in a simulation environment similar to that of DAC has not been Cubic diamond single crystal Silicon probed in literature which this manuscript addresses. Hydrostatic compression of Silicon using seven different interatomic potentials demonstrates that Tersoff(T0) performed better as compared to other potentials with regards to demonstration of phase transition. Using this Tersoff(T0) interatomic potential, molecular dynamics simulation of cubic diamond single crystal silicon has been carried out along different directions under uniaxial stress condition to determine anisotropy of the samples, if any. -tin phase could be observed for the [001] direction loading whereas Imma along with -tin phase could be observed for [011] and [111] direction loading. Amorphization is also observed for [011] direction. The results obtained in the study are based on rigorous X-ray diffraction analysis. No strain rate effects could be observed for the uniaxial loading conditions. 1. Introduction potential(SW) [19,20] for their simulations. -
Geobiology of Marine Magnetotactic Bacteria Sheri Lynn Simmons
Geobiology of Marine Magnetotactic Bacteria by Sheri Lynn Simmons A.B., Princeton University, 1999 Submitted in partial fulfillment of the requirements for the degree of Doctor of Philosophy in Biological Oceanography at the MASSACHUSETTS INSTITUTE OF TECHNOLOGY and the WOODS HOLE OCEANOGRAPHIC INSTITUTION June 2006 c Woods Hole Oceanographic Institution, 2006. Author.............................................................. Joint Program in Oceanography Massachusetts Institute of Technology and Woods Hole Oceanographic Institution May 19, 2006 Certified by. Katrina J. Edwards Associate Scientist, Department of Marine Chemistry and Geochemistry, Woods Hole Oceanographic Institution Thesis Supervisor Accepted by......................................................... Ed DeLong Chair, Joint Committee for Biological Oceanography Massachusetts Institute of Technology-Woods Hole Oceanographic Institution Geobiology of Marine Magnetotactic Bacteria by Sheri Lynn Simmons Submitted to the MASSACHUSETTS INSTITUTE OF TECHNOLOGY and the WOODS HOLE OCEANOGRAPHIC INSTITUTION on May 19, 2006, in partial fulfillment of the requirements for the degree of Doctor of Philosophy in Biological Oceanography Abstract Magnetotactic bacteria (MTB) biomineralize intracellular membrane-bound crystals of magnetite (Fe3O4) or greigite (Fe3S4), and are abundant in the suboxic to anoxic zones of stratified marine environments worldwide. Their population densities (up to 105 cells ml−1) and high intracellular iron content suggest a potentially significant role in iron -
Properties of Magnetosome Suspension Under the in Uence Of
Vol. 127 (2015) ACTA PHYSICA POLONICA A No. 2 Proceedings of the European Conference Physics of Magnetism, Pozna« 2014 Properties of Magnetosome Suspension under the Inuence of Magnetic Field A. Józefczaka;*, M. Mol£anb, Z. Rozynekc;d, T. Hornowskia, A. Skumiela, M. Timkob, J. Tóthováe, P. Kop£anskýb and B. Leszczy«skia;f aInstitute of Acoustics, Faculty of Physics, Adam Mickiewicz University, Umultowska 85, 61-614 Pozna«, Poland bInstitute of Experimental Physics, Slovak Academy of Sciences, Watsonova 47, 040 01 Ko²ice, Slovakia cInstitute of Physical Chemistry, Polish Academy of Sciences, Kasprzaka 44/52, 01-224 Warszawa, Poland dDepartment of Physics, NTNU, Høgskoleringen 5, NO-7491 Trondheim, Norway eDepartment of Physics, Technical University of Ko²ice, Park Komenského 2, 042 00 Ko²ice, Slovakia f NanoBioMedical Centre, Adam Mickiewicz University, Umultowska 85, 61-614 Pozna«, Poland The magnetotactic bacteria synthesize chains of nanosized magnetic particles that function as a compass nee- dle to navigate in the direction of the earth's magnetic eld. Magnetosomes are bacterial magnetic nanoparticles containing iron mineral crystals of magnetite or greigite, enveloped by a natural biological membrane. The objec- tive of the work is to study the inuence of magnetic eld on rheology and acoustic properties of magnetosome suspension. Experimental results show a clear eect of the external magnetic eld on the acoustic parameters such as velocity and attenuation of ultrasonic wave, and the viscosity of the suspension, arising from the biogenic magnetic particles aggregation. DOI: 10.12693/APhysPolA.127.629 PACS: 75.50.Mm, 43.35.Bf 1. Introduction Magnetosome suspension properties change under the inuence of the magnetic eld, similar to that of magnetic uid. -
19900014581.Pdf
NASA Contractor Report 4295 The Biogeochemistry of Metal Cycling Edited by Kenneth H. Nealson and Molly Nealson University of Wisconsin at Milwaukee Milwaukee, Wisconsin F. Ronald Dutcher The George Washington University Washington, D.C. Prepared for NASA Office of Space Science and Applications under Contract NASW-4324 National Aeronautics and Space Administration Office of Management Scientific and Technical Information Division 1990 Table of Contents P._gg Introduction vii ,°° Map of Oneida lake Vlll PBME Summer Schedule 1987 ix Faculty and Lecturers of the PBME 1987 Course XIII.°° Students of the PBME 1987 Course xvii I. Lecturers' Abstracts and References I Farooq Azam "Microbial Food Web Dynamics" "Mechanisms in Bacteria - Organic Matter Interactions in Aquatic Environments" Jeffrey S. Buyer "Microbial Iron Transport: Chemistry and Biochemistry" "Microbial Iron Transport: Ecology" Arthur S. Brooks "General Limnology and Primary Productivity" William C. Ghiorse "Survey of Fe/Mn-depositing (Oxidizing) Microorganisms" 10 "Lepto_hrix discoph0_: Mn Oxidation in Field and Laboratory" 12 Robert W. Howarth "Nutrient Limitation in Aquatic Ecosystems: Regulation by 13 Trace Metals" Paul E. Kepkay "Microelectrodes, Microgradients and Microbial Metabolism" 14 "In situ Dialysis: A Tool for Studying Biogeochemical Processes" 16 Edward L. Mills "Oneida Lake and Its Food Chain" 17 William S. Moore "Isotopic Tracers of Scavenging and Sedimentation" 19 "Manganese Nodules from the Deep-Sea and Oneida Lake" 21 °°° IU PRECEDING PAGE BLANK NOT FILMED James J. Morgan "Aqueous Solution, Precipitation and Redox Equilibria of 23 Manganese in Water" "Rates of Mn(ID Oxidation in Aquatic Systems: Abiotic Reactions 24 and the Importance of Surface Catalysis" James W. Murray "Mechanisms Controlling the Distribution of Trace Metals in 26 Oceans and Lakes" "Diagenesis in the Sediments of Lakes" 29 Kenneth H.