1 Identifying Dominant Recombination Mechanisms in Perovskite Solar
Total Page:16
File Type:pdf, Size:1020Kb
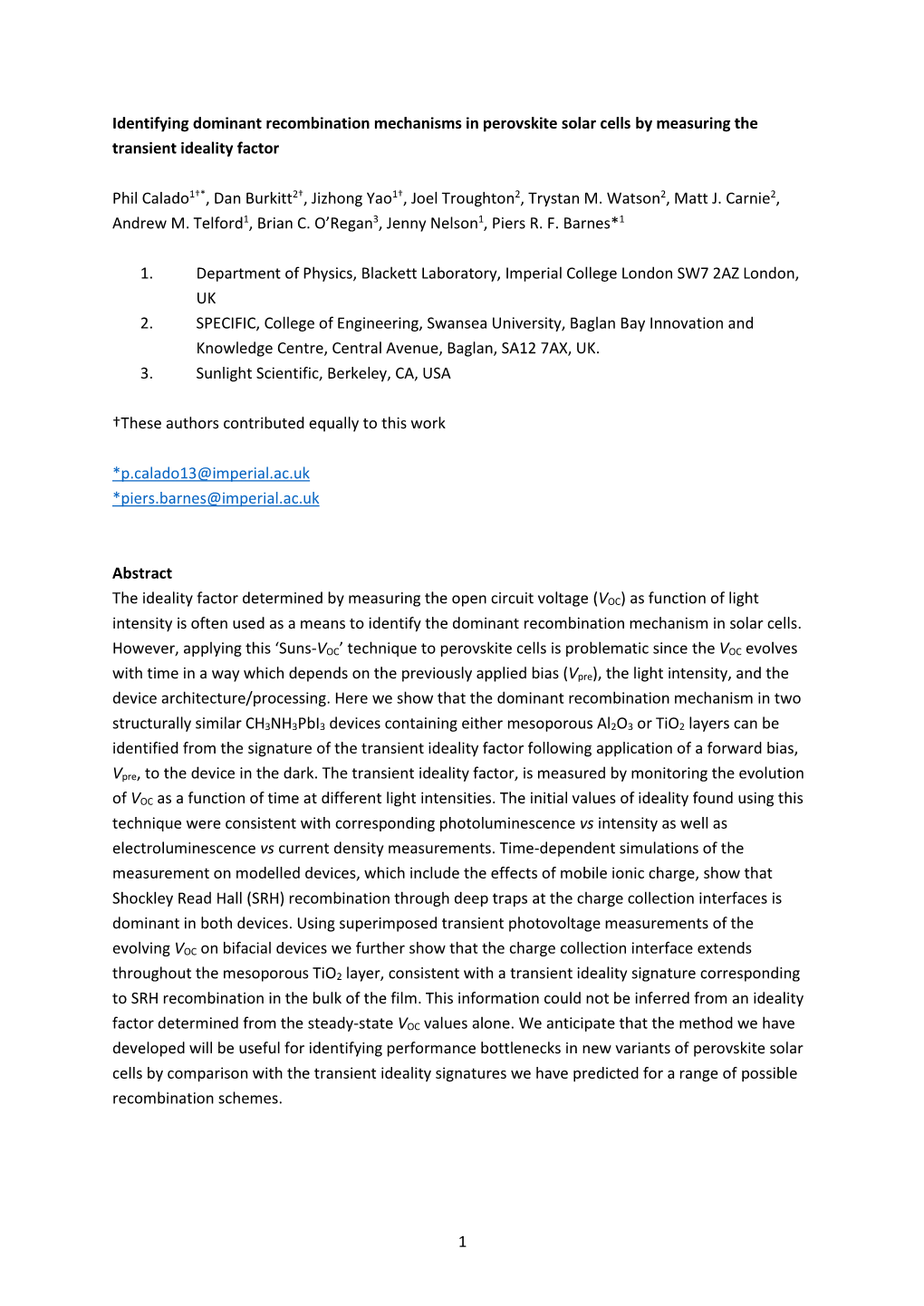
Load more
Recommended publications
-
Chapter 2 Incandescent Light Bulb
Lamp Contents 1 Lamp (electrical component) 1 1.1 Types ................................................. 1 1.2 Uses other than illumination ...................................... 2 1.3 Lamp circuit symbols ......................................... 2 1.4 See also ................................................ 2 1.5 References ............................................... 2 2 Incandescent light bulb 3 2.1 History ................................................. 3 2.1.1 Early pre-commercial research ................................ 4 2.1.2 Commercialization ...................................... 5 2.2 Tungsten bulbs ............................................. 6 2.3 Efficacy, efficiency, and environmental impact ............................ 8 2.3.1 Cost of lighting ........................................ 9 2.3.2 Measures to ban use ...................................... 9 2.3.3 Efforts to improve efficiency ................................. 9 2.4 Construction .............................................. 10 2.4.1 Gas fill ............................................ 10 2.5 Manufacturing ............................................. 11 2.6 Filament ................................................ 12 2.6.1 Coiled coil filament ...................................... 12 2.6.2 Reducing filament evaporation ................................ 12 2.6.3 Bulb blackening ........................................ 13 2.6.4 Halogen lamps ........................................ 13 2.6.5 Incandescent arc lamps .................................... 14 2.7 Electrical -
Pn JUNCTION DEVICES and LIGHT EMITTING DIODES
pn Junction Devices (© 2001 S.O. Kasap) 1 An e-Booklet pn JUNCTION DEVICES AND LIGHT EMITTING DIODES Safa Kasap Electrical Engineering Department University of Saskatchewan Canada “We consider alloyed or point contact junctions on n-type GaP. Then the light emitted with forward bias has a spectrum which is a comparatively narrow band, the position of the band depending on the impurities present in the GaP.” J.W. Allen and P.E. Gibbons (1959)1 William Shockley, (seated), John Bardeen (left) and Walter Brattain (right) invented the transistor at Bell Labs and thereby ushered in a new era of semiconductor devices. The three inventors shared the Nobel prize in 1956. (Courtesy of Bell Laboratories.) 1. pn Junction Principles 1. 1. Open Circuit Consider what happens when one side of a sample of Si is doped n-type and the other p-type as shown in Figure 1 (a). Assume that there is an abrupt discontinuity between the p and n regions which we call the metallurgical junction, M in Figure 1 (a), where the fixed (immobile) ionized donors and the free electrons (in the conduction band, CB) in the n-region and fixed ionized acceptors and holes (in the valence band, VB) in the p-region are also shown. 1 Allen and Gibbons (talking about their invention of the LED) in “Breakdown and light emission in gallium phosphide diodes”, Journal of Electronics, Vol. 7, No. 6, p. 518, December 1959. pn Junction Devices (© 2001 S.O. Kasap) 2 An e-Booklet Due to the hole concentration gradient from the p-side, where p = ppo, to the n-side where p = pno, holes diffuse towards the right and enter the n-region and recombine with the electrons (majority carriers) in this region. -
SOLAR CELLS: the Ultimate Renewable Resource – a Review
Advanced Research in Electrical and Electronic Engineering p-ISSN: 2349-5804; e-ISSN: 2349-5812 Volume 3, Issue 5, October-December, 2016, pp. 440-448 © Krishi Sanskriti Publications http://www.krishisanskriti.org/Publication.html SOLAR CELLS: The Ultimate Renewable Resource – A Review Romana Yousuf Department of Electronics and Communication Engineering, Islamic University of Science & Technology, Awantipora, J&K, India Abstract—With the growing energy demands, the need for technology which must be economically, environmentally, and socially compatible has also increased. Scientists and researchers are in struggle to utilize the solar energy. Energy delivered by sun to earth in one hour is equal to the energy consumed by the people in one year. In recent years solar cell technology has achieved tremendous growth as sustainable source of energy. The fabrication of solar cells has passed through a large number of improvement steps from one generation to another. Silicon based solar cells were the first developed. Further development in solar cells results in enhanced cell efficiency. The progress is basically hindered by the cost and efficiency. In order to choose the right solar cell for a specific geographic location, we are required to understand fundamental mechanisms and functions of several solar technologies that are widely studied. In this paper, we have reviewed the operation, structure, efficiency and models of the conventionally used solar cells along with progressive development in the solar cell research from one generation to other. 1. INTRODUCTION Since the last decade, the world is developing at a pace like never before. An important factor behind this evolution is the recent advancements in the field of the energy generation technology. -
Basic Semiconductor Material Science and Solid State Physics
Electrical Behavior of pn-Junctions To consider what happens electrically in a pn-junction, one can, again, consider a thought experiment. Suppose that two blocks of semiconductor of opposite extrinsic doping, i.e., one block is n-type and one is p-type, are initially separated widely. If these blocks are then joined, a net transfer of carriers from one block to the other must occur in order to establish equilibrium. Obviously, this situation is similar to the “simpler” cases of a metal-semiconductor contact and an MOS capacitor. Furthermore, just as in these previous cases, if the whole system is to be in equilibrium, then the Fermi level must be constant throughout the entire combined volume of the two blocks. It comes as no surprise that this requires that valence and conduction bands must become bent in the region of the junction. This situation is illustrated below: Evac EC E Fn E F Ei E Fp EV n-type p-type pn-junction Fig. 55: Band diagrams for an unbiased pn-junction The position of the junction is determined as the point that the intrinsic Fermi level and the actual Fermi level intersect. Of course, this defines the exact position at which the semiconductor changes from one type to the other, i.e., it is intrinsic at the junction. Moreover, even though this structure is hypothetically constructed by joining separate blocks of material, the physical mechanism used to form a pn-junction is immaterial to its electrical behavior. Obviously, in practice dopant diffusion processes can be used to form pn-junctions intentionally. -
PN Junctions This Worksheet and All Related Files Are Licensed Under The
PN junctions This worksheet and all related files are licensed under the Creative Commons Attribution License, version 1.0. To view a copy of this license, visit http://creativecommons.org/licenses/by/1.0/, or send a letter to Creative Commons, 559 Nathan Abbott Way, Stanford, California 94305, USA. The terms and conditions of this license allow for free copying, distribution, and/or modification of all licensed works by the general public. Resources and methods for learning about these subjects (list a few here, in preparation for your research): 1 Questions Question 1 When ”P” and ”N” type semiconductor pieces are brought into close contact, free electrons from the ”N” piece will rush over to fill holes in the ”P” piece, creating a zone on both sides of the contact region devoid of charge carriers. What is this zone called, and what are its electrical characteristics? file 00715 Question 2 What happens to the thickness of the depletion region in a PN junction when an external voltage is applied to it? file 00909 2 Question 3 Shown here are two energy diagrams: one for a ”P” type semiconducting material and another for an ”N” type. PN Conduction band Ef "Acceptor" holes "Donor" electrons Ef Valence band Increasing electron energy Next is an energy diagram showing the initial state when these two pieces of semiconducting material are brought into contact with each other. This is known as a flatband diagram: "Flatband" diagram -- temporary state! PN Conduction band Ef2 "Acceptor" holes "Donor" electrons Ef1 Valence band The state represented by the ”flatband” diagram is most definitely a temporary one. -
Light-Emitting Diodes Second Edition
This page intentionally left blank LIGHT-EMITTING DIODES SECOND EDITION Revised and fully updated, the Second Edition of this textbook offers a comprehensive explanation of the technology and physics of light-emitting diodes (LEDs) such as infrared, visible-spectrum, ultraviolet, and white LEDs made from III–V semiconductors. The elementary properties of LEDs such as electrical and optical characteristics are reviewed, followed by the analysis of advanced device structures. With nine additional chapters, the treatment of LEDs has been vastly expanded, including new material on device packaging, reflectors, UV LEDs, III–V nitride materials, solid-state sources for illumination applications, and junction temperature. Radiative and non-radiative recombination dynamics, methods for improving light extraction, high-efficiency and high- power device designs, white-light emitters with wavelength-converting phosphor materials, optical reflectors, and spontaneous recombination in resonant-cavity structures, are dis- cussed in detail. Fields related to solid-state lighting such as human vision, photometry, colorimetry, and color rendering are covered beyond the introductory level provided in the first edition. The applications of infrared and visible-spectrum LEDs in silica fiber, plas- tic fiber, and free-space communication are also discussed. Semiconductor material data, device design data, and analytic formulae governing LED operation are provided. With exercises, solutions and illustrative examples, this textbook will be of interest to scientists and engineers working on LEDs, and to graduate students in electrical engineering, applied physics, and materials science. Additional resources for this title are available online at www.cambridge.org/ 9780521865388. E. Fred Schubert received his Ph.D. degree with Honors in Electrical Engineering from University of Stuttgart in 1986 and is currently a Wellfleet Senior Constellation Pro- fessor of the Future Chips Constellation at Rensselaer Polytechnic Institute. -
Understanding the Current-Voltage Characteristics of Industrial Crystalline Silicon Solar Cells by Considering Inhomogeneous Current Distributions Invited Paper O
OPTO−ELECTRONICS REVIEW 21(3), 259–282 DOI: 10.2478/s11772−013−0095−5 Understanding the current-voltage characteristics of industrial crystalline silicon solar cells by considering inhomogeneous current distributions Invited Paper O. BREITENSTEIN* Max Planck Institute of Microstructure Physics, 2 Weinberg, D−06120 Halle, Germany Solar cells made from multi− or mono−crystalline silicon wafers are the base of today’s photovoltaics industry. These devices are essentially large−area semiconductor p−n junctions. Technically, solar cells have a relatively simple structure, and the theory of p−n junctions was established already decades ago. The generally accepted model for describing them is the so−called two−diode model. However, the current−voltage characteristics of industrial solar cells, particularly of that made from multi−crystalline silicon material, show significant deviations from established diode theory. These deviations regard the forward and the reverse dark characteristics as well as the relation between the illuminated characteristics to the dark ones. In the recent years it has been found that the characteristics of industrial solar cells can only be understood by taking into account local inhomogeneities of the dark current flow. Such inhomogeneities can be investigated by applying lock−in thermography techniques. Based on these and other investigations, meanwhile the basic properties of industrial silicon solar cells are well understood. This contribution reviews the most important experimental results leading to the present state of physical understanding of the dark and illuminated characteristics of multi−crystalline industrial solar cells. This analysis should be helpful for the continuing process of optimizing such cells for further increasing their energy conversion efficiency. -
A Simple Method for Power Diodes Parameter Measurement
ISSN (Print) : 2320 – 3765 ISSN (Online): 2278 – 8875 International Journal of Advanced Research in Electrical, Electronics and Instrumentation Engineering (An ISO 3297: 2007 Certified Organization) Vol. 5, Issue 8, August 2016 A Simple Method for Power Diodes Parameter Measurement Farzin Asadi1, Nurettin Abut2 Student, Kocaeli University, Kocaeli, Turkey1 Professor, Dept. of Electrical Engineering, Kocaeli University, Kocaeli, Turkey2 ABSTRACT: Switches are heart of power electronics. Plenty of switches are available today. Diode is one of the most wide spread switches which has a lot of application in power electronic and small signal electronics. Diode, has a non- linear I-V characteristics. Diode I-V relation is governed by Shockley diode equation. Two parameters are in Shockley equation: Reverse saturation current(Is) and emission coefficient(n). This parameters are vary from device to device. Reverse saturation current is very small for modern silicon diodes, generally in the range 10-12 A to 10-8A, very hard to measure it directly. A simple method for measuring these parameters is proposed here. No expensive measurement device is required. This method can also be used for fault detection of power diodes. Laboratory results shows the success of given method. KEYWORDS: Emission coefficient, Power diodes, Reverse saturation current, Shockley equation. I.INTRODUTION Power electronics is the application of solid-state electronics to the control and conversion of electric power. power conversion systems can be classified according to the type of the input and output power: AC to DC (rectifier), DC to AC (inverter), DC to DC (DC-to-DC converter), AC to AC (AC-to-AC converter). -
Comparative Analysis Between Single Diode and Double Diode Model of PV Cell: Concentrate Different Parameters Effect on Its Efficiency
Journal of Power and Energy Engineering, 2016, 4, 31-46 Published Online March 2016 in SciRes. http://www.scirp.org/journal/jpee http://dx.doi.org/10.4236/jpee.2016.43004 Comparative Analysis between Single Diode and Double Diode Model of PV Cell: Concentrate Different Parameters Effect on Its Efficiency Tanvir Ahmad*, Sharmin Sobhan, Md. Faysal Nayan Department of Electrical and Electronic Engineering, Ahsanullah University of Science and Technology, Dhaka, Bangladesh Received 3 February 2016; accepted 28 March 2016; published 31 March 2016 Copyright © 2016 by authors and Scientific Research Publishing Inc. This work is licensed under the Creative Commons Attribution International License (CC BY). http://creativecommons.org/licenses/by/4.0/ Abstract This research appraises comparative analysis between single diode and double diode model of photovoltaic (PV) solar cells to enhance the conversion efficiency of power engendering PV solar systems. Single diode model is simple and easy to implement, whereas double diode model has better accuracy which acquiesces for more precise forecast of PV systems performance. Explora- tion is done on the basis of simulation results and MATLAB tool is used to serve this purpose. Si- mulations are performed by varying distinct model parameters such as solar irradiance, temper- ature, value of parasitic resistances, ideality factor of diode and number of series and parallel connected solar cells used to assemble PV array. Conspicuous demonstration is executed to ana- lyze effects of these specifications on the efficiency curve and power vs. voltage output characte- ristics of PV cell for specified models. Keywords Photovoltaic Cell, Single Diode Model, Double Diode Model, Efficiency, Simulation 1. -
Semiconductor Diodes
Semiconductor diodes A modern semiconductor diode is made of a crystal of semiconductor like silicon that has impurities added to it to create a region on one side that contains negative charge carriers (electrons), called n-type semiconductor, and a region on the other side that contains positive charge carriers (holes), called p-type semiconductor. The diode's terminals are attached to each of these regions. The boundary within the crystal between these two regions, called a PN junction, is where the action of the diode takes place. The crystal conducts “electron current” in a direction from the n-type side (called the cathode), to the p-type side (called the anode), but not in the opposite direction or for those familiar with “conventional current” in a direction from the p-type side (called the anode) to the n-type side (called the cathode), but not in the opposite direction. Another type of semiconductor diode, the Schottky diode, is formed from the contact between a metal and a semiconductor rather than by a p-n junction. Rectifier A rectifier is an electrical device that converts alternating current (AC) to direct current (DC), a process known as rectification. Rectifiers have many uses including as components of power supplies and as detectors of radio signals. Rectifiers may be made of solid state diodes, vacuum tube diodes, mercury arc valves, and other components. A device which performs the opposite function (converting DC to AC) is known as an inverter. When only one diode is used to rectify AC (by blocking the negative or positive portion of the waveform), the difference between the term diode and the term rectifier is merely one of usage, i.e., the term rectifier describes a diode that is being used to convert AC to DC. -
Towards Deeper Comprehension in Higher Engineering Education: Rethinking "In Theory Yes, but Not in Practice"
European Journal of Science and Mathematics Education Vol. 3, No. 4, 2015, 390‐407 Towards deeper comprehension in higher engineering education: rethinking "in theory yes, but not in practice" Aki Korpela1, Timo Tarhasaari2, Lauri Kettunen2, Risto Mikkonen2 and Hanna Kinnari- Korpela1 1 Department of Electrical Engineering, Tampere University of Applied Sciences, Tampere, Finland 2 Electromagnetics, Tampere University of Technology, Tampere, Finland For correspondence: [email protected] Abstract In the economic pressure of recent decades, many universities have invested in increasing the number of accessed and graduated students. However, this has led to another problem: there is not enough higher learning in higher education. According to generally accepted view, the problem lies in the eagerness of overplaying immediate skills in engineering education. To manage with the situation we propose that special attention is paid to logical reasoning behind the models employed in elementary engineering education. The idea is based on the hierarchical nature of physics, which enables a consistent method to avoid the risk of overplaying the immediate skills. In this paper, we seek for higher learning by proposing such a method and presenting a case study of solar electricity. When modelling the nature, on each level of hierarchy certain laws of physics lay the foundation for explanations there. We call them the cornerstones of modelling. Due to the hierarchical nature of physics, the cornerstones on a certain level of hierarchy are only built from the ones on the lower level of higher abstraction. When we stand above the cornerstones, they appear as unchallenged rules. But most importantly, if we take a hierarchical step towards fundamental models and look underneath the cornerstones, instead of unchallenged rules, they appear as testable properties. -
This File Has Been Cleaned of Potential Threats. If You Confirm That the File Is Coming from a Trusted Source, You Can Send
This file has been cleaned of potential threats. If you confirm that the file is coming from a trusted source, you can send the following SHA-256 hash value to your admin for the original file. be221cb64aa6dff78d3dc62e0af11f7ca037eb2235c4ff20388689ac732d0f60 To view the reconstructed contents, please SCROLL DOWN to next page. Solved Questions Semiconductor Principle Questions (1) Don’t just sit there! Build something!! Learning to mathematically analyze circuits requires much study and practice. Typically, students practice by working through lots of sample problems and checking their answers against those provided by the textbook or the instructor. While this is good, there is a much better way. You will learn much more by building and analyzing real circuits, letting your test equipment provide the “answers” instead of a book or another person. For successful circuit-building exercises, follow these steps: 1. Carefully measure and record all component values prior to circuit construction, choosing resistor values high enough to make damage to any active components unlikely. 2. Draw the schematic diagram for the circuit to be analyzed. 3. Carefully build this circuit on a breadboard or other convenient medium. 4. Check the accuracy of the circuit’s construction, following each wire to each connection point, and verifying these elements one- by-one on the diagram. 5. Mathematically analyze the circuit, solving for all voltage and current values. 6. Carefully measure all voltages and currents, to verify the accuracy of your analysis. 7. If there are any substantial errors (greater than a few percent), carefully check your circuit’s construction against the diagram, then re-calculate the values and re-measure.