The Effect of Nitrogen on Rhizobacteria Associated with Wheat Shoot Productivity
Total Page:16
File Type:pdf, Size:1020Kb
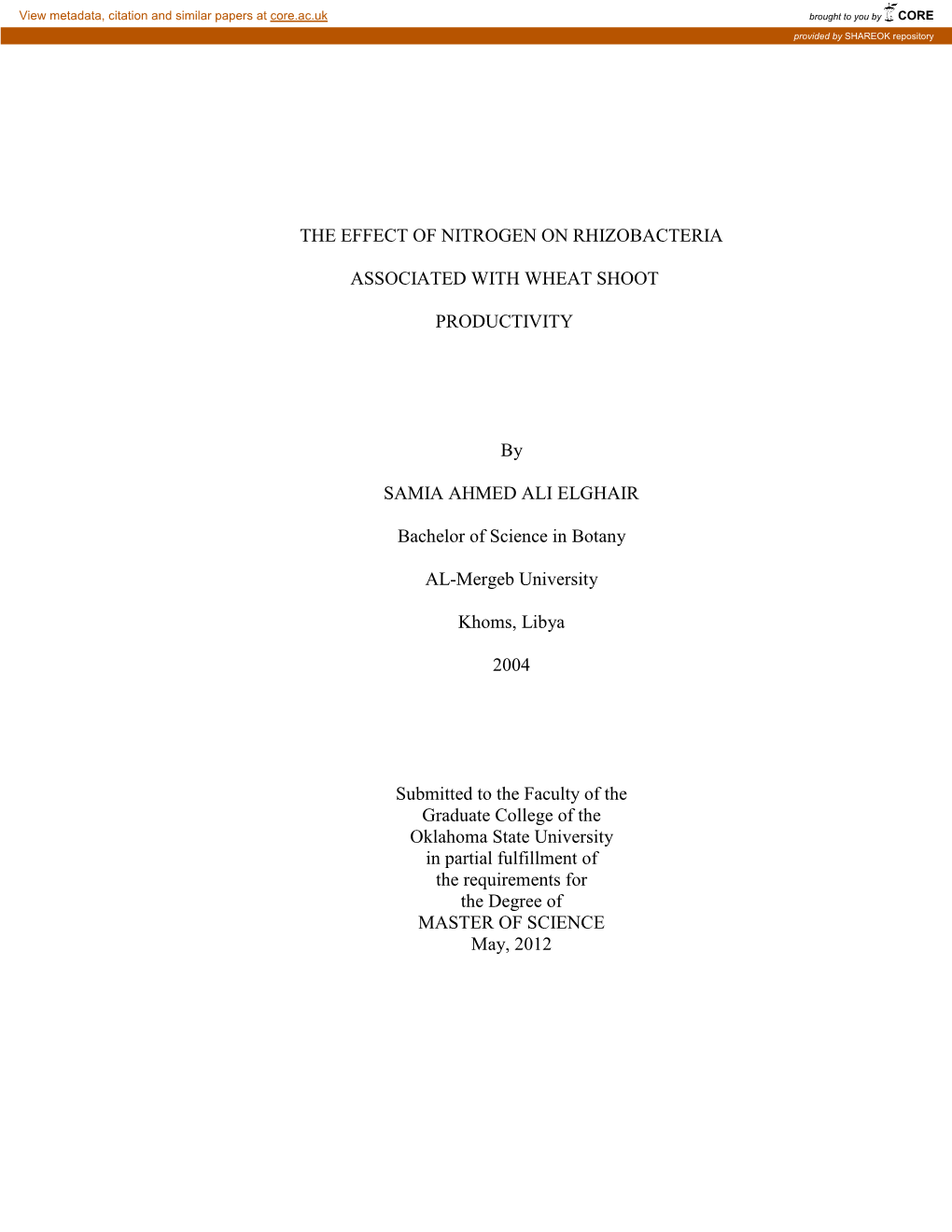
Load more
Recommended publications
-
Stability and Succession of the Rhizosphere Microbiota Depends Upon Plant Type and Soil Composition
The ISME Journal (2015) 9, 2349–2359 © 2015 International Society for Microbial Ecology All rights reserved 1751-7362/15 www.nature.com/ismej ORIGINAL ARTICLE Stability and succession of the rhizosphere microbiota depends upon plant type and soil composition Andrzej Tkacz1,2, Jitender Cheema1,3, Govind Chandra1, Alastair Grant4 and Philip S Poole1,2 1Department of Molecular Microbiology, John Innes Centre, Norwich Research Park, Norwich, UK; 2Department of Plant Sciences, Oxford University, Oxford, UK; 3Department of Computational and Systems Biology, John Innes Centre, Norwich Research Park, Norwich, UK and 4Earth and Life Systems Alliance, The School of Environmental Sciences, University of East Anglia, Norwich, UK We examined succession of the rhizosphere microbiota of three model plants (Arabidopsis, Medicago and Brachypodium) in compost and sand and three crops (Brassica, Pisum and Triticum) in compost alone. We used serial inoculation of 24 independent replicate microcosms over three plant generations for each plant/soil combination. Stochastic variation between replicates was surprisingly weak and by the third generation, replicate microcosms for each plant had communities that were very similar to each other but different to those of other plants or unplanted soil. Microbiota diversity remained high in compost, but declined drastically in sand, with bacterial opportunists and putative autotrophs becoming dominant. These dramatic differences indicate that many microbes cannot thrive on plant exudates alone and presumably also require carbon sources and/or nutrients from soil. Arabidopsis had the weakest influence on its microbiota and in compost replicate microcosms converged on three alternative community compositions rather than a single distinctive community. Organisms selected in rhizospheres can have positive or negative effects. -
The RDP-II Backbone Tree for Release 8.0. the Tree Was Inferred from a Distance Matrix Generated in PAUP* with the Weighbor (Weighted Neighbor Joining) Algorithm
Methylococcus capsulatus ACM 1292 (T) Oceanospirillum linum ATCC 11336 (T) Halomonas halodenitrificans ATCC 13511 (T) Legionella lytica PCM 2298 (T) Francisella tularensis subsp. tularensis ATCC 6223 (T) Coxiella burnetii Q177 Moraxella catarrhalis ATCC 25238 (T) Pseudomonas fluorescens IAM 12022 (T) Piscirickettsia salmonis LF-89 (T) Thiothrix nivea DSM 5205 (T) Allochromatium minutissimum DSM 1376 (T) Alteromonas macleodii IAM 12920 (T) Aeromonas salmonicida subsp. smithia CCM 4103 (T) Pasteurella multocida NCTC 10322 (T) Enterobacter nimipressuralis LMG 10245 (T) Vibrio vulnificus ATCC 27562 (T) Ectothiorhodospira mobilis DSM237 (T) Xanthomonas campestris LMG 568 (T) Cardiobacterium hominis ATCC 15826 (T) Methylophilus methylotrophus ATCC 53528 (T) Rhodocyclus tenuis DSM 109 (T) Hydrogenophilus thermoluteolus TH-1 (T) Neisseria gonorrhoeae NCTC 8375 (T) Comamonas testosteroni ATCC 11996 (T) Nitrosospira multiformis ATCC 25196 (T) Spirillum volutans ATCC 19554 (T) Burkholderia glathei LMG 14190 (T) Alcaligenes defragrans DSM 12141 (T) Oxalobacter formigenes ATCC 35274 (T) Acetobacter oboediens DSM 11826 (T) clone CS93 PROTEOBACTERIA Caedibacter caryophilus 221 (T) Rhodobacter sphaeroides ATCC 17023 (T) Rickettsia rickettsii ATCC VR-891 (T) Ehrlichia risticii ATCC VR-986 (T) Sphingomonas paucimobilis GIFU 2395 (T) Caulobacter fusiformis ATCC 15257 (T) Rhodospirillum rubrum ATCC 11170 (T) Brucella melitensis ATCC 23459 (T) Rhizobium tropici IFO 15247 (T) Bartonella vinsonii subsp. vinsonii ATCC VR-152 (T) Phyllobacterium myrsinacearum -
Enhanced Drought Tolerance Through Plant Growth Promoting
ENHANCED DROUGHT TOLERANCE THROUGH PLANT GROWTH PROMOTING RHIZOBACTERIA AND MICROBIOME ENGINEERING APPLICATIONS A Dissertation by MICHAEL DONOVAN JOCHUM JR. Submitted to the Office of Graduate and Professional Studies of Texas A&M University in partial fulfillment of the requirements for the degree of DOCTOR OF PHILOSOPHY Chair of Committee, Young-Ki Jo Committee Members, Elizabeth Pierson Heather Wilkson Leland Pierson III Head of Department, Leland Pierson III May 2019 Major Subject: Plant Pathology Copyright 2019 Michael Jochum Jr. ABSTRACT Water is a major limiting resource in agriculture worldwide, restricting crop yields in approximately 70 percent of arable farmlands. My goal was to alleviate drought stress in grasses using plant growth-promoting rhizobacteria (PGPR) and host-mediated microbiome engineering (HMME) of the rhizosphere. In the summers of 2016 and 2017, we collected bermudagrass rhizospheres from El Paso, TX for PGPR bioprospecting. Two novel isolates, Bacillus sp. (12D6) and Enterobacter sp. (16i) , were shown to delay the onset of drought stress in wheat (Triticum aestivum subsp. aestivum cultivar TAM 111) and maize (Zea maize cultivar B73) seedlings. Roots inoculated with these PGPR resulted in statistically significant alterations in root system architecture traits associated with drought tolerance in a host-specific manner. In the second part of this study, I employed host-mediated microbiome engineering to confer a generational increase in drought tolerance of wheat seedlings. In this host-centric artificial selection process, the wheat rhizosphere was sub-selected based on host phenotypic tolerance after a prolonged water deficit. After six rounds of microbiome engineering, seedlings growing in the engineered microbiome withstood an additional 5 days of water deficit compared to the initial microbiome. -
Nitrogen Fixing and Phosphate Mineralizing Bacterial Communities in Sweet Potato Rhizosphere Show a Genotype-Dependent Distribution
diversity Article Nitrogen Fixing and Phosphate Mineralizing Bacterial Communities in Sweet Potato Rhizosphere Show a Genotype-Dependent Distribution Joana Montezano Marques 1, Jackeline Rossetti Mateus 2, Thais Freitas da Silva 2, Camila Rattes de Almeida Couto 2, Arie Fitzgerald Blank 3 and Lucy Seldin 2,* 1 Instituto de Ciências Biológicas, Centro de Genômica e Biologia de Sistemas, Universidade Federal do Pará, Av. Augusto Corrêa, 01, Guamá, CEP 66.075-110, Belém, PA, Brazil; [email protected] 2 Instituto de Microbiologia Paulo de Góes (IMPPG), Universidade Federal do Rio de Janeiro, Centro de Ciências da Saúde, Bloco I, Ilha do Fundão, CEP 21941-590, Rio de Janeiro, RJ, Brazil; [email protected] (J.R.M.); [email protected] (T.F.d.S.); [email protected] (C.R.d.A.C.) 3 Departamento de Engenharia Agronômica, Universidade Federal de Sergipe, Av. Marechal Rondon S/N, CEP 49100-000, São Cristóvão, SE, Brazil; [email protected] * Correspondence: [email protected]; Tel.: +55-213-938-6741; Fax: +55-212-560-8344 Received: 23 October 2019; Accepted: 28 November 2019; Published: 3 December 2019 Abstract: We hypothesize that sweet potato genotypes can influence the bacterial communities related to phosphate mineralization and nitrogen fixation in the rhizosphere. Tuberous roots of field-grown sweet potato from genotypes IPB-149, IPB-052, and IPB-137 were sampled three and six months after planting. The total community DNA was extracted from the rhizosphere and analyzed by Polymerase Chain Reaction-Denaturing Gradient Gel Electrophoresis (PCR-DGGE) and quantitative real-time PCR (qPCR), based on the alkaline phosphatase coding gene (alp gene) and on the nitrogenase coding gene (nifH gene). -
Effectiveness of Exopolysaccharides and Biofilm Forming Plant Growth Promoting Rhizobacteria on Salinity Tolerance of Faba Bean (Vicia Faba L.)
Vol. 12(17), pp. 399-404, 7 May, 2018 DOI: 10.5897/AJMR2018.8822 Article Number: 2E927E057083 ISSN 1996-0808 Copyright © 2018 Author(s) retain the copyright of this article African Journal of Microbiology Research http://www.academicjournals.org/AJMR Full Length Research Paper Effectiveness of exopolysaccharides and biofilm forming plant growth promoting rhizobacteria on salinity tolerance of faba bean (Vicia faba L.) Alaa Fathalla Mohammed Department of Agric. Botany, Faculty of Agriculture, Suez Canal University, Ismailia, Egypt. Received 22 February, 2018; Accepted 27 March, 2018 This study aimed to investigate the production of biofilm and exopolysaccharides by plant growth promoting rhizobacteria (PGPR) under different salt concentrations. In this study, the activity of biofilm formation and exopolysaccharides production by 20 strains of PGPR which previously isolated and identified from root samples of different crops were determined under different salt concentrations. Out of 20 strains, only 12 PGPR strains have the ability to form biofilm at 0.0, 50, 100 and 150 mM NaCl concentration. PGPR strains with the highest activity of biofilm formation and exopolysaccharides production were selected to check them on the faba bean plants under different concentration of salt stress. Inoculation with PGPR strains increased plant growth at higher level of salt concentrations compared with their corresponding uninoculated ones. The strain Pseudomonas anguilliseptica SAW 24 showed the highest activity of biofilm formation and exopolysaccharides production at different NaCl concentrations furthermore, gave the highest records of plant height (cm), fresh and dry weight (g/plant) of faba bean plants. The activities of biofilm formation and exopolysaccharides production of plant growth promoting bacteria enhance faba bean plants against different salt concentrations. -
The Influence of Sodium Chloride on the Performance of Gammarus Amphipods and the Community Composition of Microbes Associated with Leaf Detritus
THE INFLUENCE OF SODIUM CHLORIDE ON THE PERFORMANCE OF GAMMARUS AMPHIPODS AND THE COMMUNITY COMPOSITION OF MICROBES ASSOCIATED WITH LEAF DETRITUS By Shelby McIlheran Leaf litter decomposition is a fundamental part of the carbon cycle and helps support aquatic food webs along with being an important assessment of the health of rivers and streams. Disruptions in this organic matter breakdown can signal problems in other parts of ecosystems. One disruption is rising chloride concentrations. Chloride concentrations are increasing in many rivers worldwide due to anthropogenic sources that can harm biota and affect ecosystem processes. Elevated chloride concentrations can lead to lethal or sublethal impacts. While many studies have shown that excessive chloride uptake impacts health (e.g. lowered respiration and growth rates) in a wide variety of aquatic organisms including microbes and benthic invertebrates). The impacts of high chloride concentrations on decomposers are less well understood. My research objective was to assess how increasing chloride concentrations affect the performance and diversity of decomposer organisms in freshwater systems. I experimentally manipulated chloride concentrations in microcosms containing leaves colonized by microbes or containing leaves, microbes and amphipods. Respiration rate, decomposition, and community composition of the microbes were measured along with the amphipod growth rate, egestion rate, and mortality. Elevated chloride concentration did not impact microbial respiration rates or leaf decomposition, but had large impacts on bacteria community composition. It did cause a decrease in instantaneous growth rate, and 100% mortality in the highest amphipod chloride treatment, but amphipod egestion rate was not significantly affected. The results of my research suggest that the widespread increases in chloride concentrations in rivers will have an impact on decomposer communities in these systems. -
Antibus Revised Thesis 11-16 For
Molecular and Cultivation-based Characterization of Ancient Algal Mats from the McMurdo Dry Valleys, Antarctica A thesis submitted to Kent State University in partial fulfillment of the requirements for the degree of Master of Science by Doug Antibus December, 2009 Thesis written by Doug Antibus B.S., Kent State University, 2007 M.S., Kent State University, 2009 Approved by Dr. Christopher B. Blackwood Advisor Dr. James L. Blank Chair, Department of Biological Sciences Dr. Timothy Moerland Dean, College of Arts and Sciences iii TABLE OF CONTENTS LIST OF TABLES………………………………………………………………………..iv LIST OF FIGURES ……………………………………………………………………...vi ACKNOWLEDGEMENTS…………………………………………………………......viii CHAPTER I: General Introduction……………………………………………………….1 CHAPTER II: Molecular Characterization of Ancient Algal Mats from the McMurdo Dry Valleys, Antarctica: A Legacy of Genetic Diversity Introduction……………………………………………………………....22 Results and Discussion……………………………………………..……27 Methods…………………………………………………………………..51 Literature Cited…………………………………………………………..59 CHAPTER III: Recovery of Viable Bacteria from Ancient Algal Mats from the McMurdo Dry Valleys, Antarctica Introduction………………………………………………..……………..78 Methods…………………………………………………………………..80 Results……………………………………………………………...…….88 Discussion…………………………………………………………...….106 Literature Cited………………………………………………………....109 CHAPTER IV: General Discussion…………………………………………………….120 iii LIST OF TABLES Chapter II: Molecular Characterization of Ancient Algal Mats from the McMurdo Dry Valleys, Antarctica: A Legacy of Genetic Diversity -
Effect of Biofilm Forming Plant Growth Promoting Rhizobacteria On
View metadata, citation and similar papers at core.ac.uk brought to you by CORE provided by Elsevier - Publisher Connector Annals of Agricultural Science (2016) 61(2), 217–227 HOSTED BY Faculty of Agriculture, Ain Shams University Annals of Agricultural Science www.elsevier.com/locate/aoas Effect of biofilm forming plant growth promoting rhizobacteria on salinity tolerance in barley Wedad A. Kasim a,*, Reda M. Gaafar a, Rania M. Abou-Ali b, Mohamed N. Omar c, Heba M. Hewait c a Botany Department, Faculty of Science, Tanta University, Tanta, Egypt b Department of Nucleic Acid and Protein Structure, Agriculture Genetic Engineering Research Institute, Agriculture Research Centre, Giza, Egypt c Department of Microbiology, Soil, Water and Environment Research Institute, Agriculture Research Centre, Giza, Egypt Received 21 May 2016; revised 13 July 2016; accepted 13 July 2016 Available online 3 September 2016 KEYWORDS Abstract Formation of biofilm under varying stress conditions is a significant strategy adopted by Bacillus amyloliquifaciens; bacterial strains for their successful survival in plant rhizosphere. In this study, the activity of bio- Barley; film formation of 20 isolates and strains of plant growth promoting rhizobacteria (PGPR) was Biofilm; determined under different salt concentrations. The results indicated that all of the 20 PGPRs have Plant growth promoting rhi- the activity of biofilm formation under 0.0, 250, 500 or 1000 mM NaCl which was increased with zobacteria; increasing salt concentration. PGPR strains with the highest activity of biofilm formation were Salinity selected and used to coat barley grains. The coated grains were sown in clay/sandy soil and left to grow for 25 days. -
Inter-Domain Horizontal Gene Transfer of Nickel-Binding Superoxide Dismutase 2 Kevin M
bioRxiv preprint doi: https://doi.org/10.1101/2021.01.12.426412; this version posted January 13, 2021. The copyright holder for this preprint (which was not certified by peer review) is the author/funder, who has granted bioRxiv a license to display the preprint in perpetuity. It is made available under aCC-BY-NC-ND 4.0 International license. 1 Inter-domain Horizontal Gene Transfer of Nickel-binding Superoxide Dismutase 2 Kevin M. Sutherland1,*, Lewis M. Ward1, Chloé-Rose Colombero1, David T. Johnston1 3 4 1Department of Earth and Planetary Science, Harvard University, Cambridge, MA 02138 5 *Correspondence to KMS: [email protected] 6 7 Abstract 8 The ability of aerobic microorganisms to regulate internal and external concentrations of the 9 reactive oxygen species (ROS) superoxide directly influences the health and viability of cells. 10 Superoxide dismutases (SODs) are the primary regulatory enzymes that are used by 11 microorganisms to degrade superoxide. SOD is not one, but three separate, non-homologous 12 enzymes that perform the same function. Thus, the evolutionary history of genes encoding for 13 different SOD enzymes is one of convergent evolution, which reflects environmental selection 14 brought about by an oxygenated atmosphere, changes in metal availability, and opportunistic 15 horizontal gene transfer (HGT). In this study we examine the phylogenetic history of the protein 16 sequence encoding for the nickel-binding metalloform of the SOD enzyme (SodN). A comparison 17 of organismal and SodN protein phylogenetic trees reveals several instances of HGT, including 18 multiple inter-domain transfers of the sodN gene from the bacterial domain to the archaeal domain. -
Impact of Introduction of Arbuscular Mycorrhizal Fungi on the Root Microbial Community in Agricultural Fields
Microbes Environ. Vol. 34, No. 1, 23-32, 2019 https://www.jstage.jst.go.jp/browse/jsme2 doi:10.1264/jsme2.ME18109 Impact of Introduction of Arbuscular Mycorrhizal Fungi on the Root Microbial Community in Agricultural Fields TURGUT YIGIT AKYOL1, RIEKO NIWA2, HIDEKI HIRAKAWA3, HayatO MARUyama4, TAKUMI SatO5, TAKAE SUZUKI6, AyaKO FUKUNAGA7, TAKASHI SatO8, SHIGENOBU YOSHIDA2, KEItaRO TAWARaya5, MASANORI SAITO6,9, TatsUHIRO EZAWA4, and SHUSEI SatO1* 1Graduate School of Life Sciences, Tohoku University, Sendai 980–8577, Japan; 2Central Region Agricultural Research Center, National Agriculture and Food Research Organization (NARO), 2–1–18 Kannondai, Tsukuba 305–8666, Japan; 3Kazusa DNA Research Institute, Kisarazu 292–0818, Japan; 4Graduate School of Agriculture, Hokkaido University, Sapporo 060–8589, Japan; 5Faculty of Agriculture, Yamagata University, Tsuruoka 997–8555, Japan; 6Field Science Center, Graduate School of Agriculture, Tohoku University, Osaki 989–6711, Japan; 7Western Region Agricultural Research Center, NARO, Ayabe 623–0035, Japan; 8Faculty of Bioresource Sciences, Akita Prefectural University, Akita 010–0195, Japan; and 9Department of Innovation Research, Japan Science and Technology Agency, Tokyo, 102–0076, Japan (Received July 31, 2018—Accepted October 22, 2018—Published online December 22, 2018) Arbuscular mycorrhizal (AM) fungi are important members of the root microbiome and may be used as biofertilizers for sustainable agriculture. To elucidate the impact of AM fungal inoculation on indigenous root microbial communities, we used high-throughput sequencing and an analytical pipeline providing fixed operational taxonomic units (OTUs) as an output to investigate the bacterial and fungal communities of roots treated with a commercial AM fungal inoculum in six agricultural fields. AM fungal inoculation significantly influenced the root microbial community structure in all fields. -
Bibliography
Bibliography Abella, C.A., X.P. Cristina, A. Martinez, I. Pibernat and X. Vila. 1998. on moderate concentrations of acetate: production of single cells. Two new motile phototrophic consortia: "Chlorochromatium lunatum" Appl. Microbiol. Biotechnol. 35: 686-689. and "Pelochromatium selenoides". Arch. Microbiol. 169: 452-459. Ahring, B.K, P. Westermann and RA. Mah. 1991b. Hydrogen inhibition Abella, C.A and LJ. Garcia-Gil. 1992. Microbial ecology of planktonic of acetate metabolism and kinetics of hydrogen consumption by Me filamentous phototrophic bacteria in holomictic freshwater lakes. Hy thanosarcina thermophila TM-I. Arch. Microbiol. 157: 38-42. drobiologia 243-244: 79-86. Ainsworth, G.C. and P.H.A Sheath. 1962. Microbial Classification: Ap Acca, M., M. Bocchetta, E. Ceccarelli, R Creti, KO. Stetter and P. Cam pendix I. Symp. Soc. Gen. Microbiol. 12: 456-463. marano. 1994. Updating mass and composition of archaeal and bac Alam, M. and D. Oesterhelt. 1984. Morphology, function and isolation terial ribosomes. Archaeal-like features of ribosomes from the deep of halobacterial flagella. ]. Mol. Biol. 176: 459-476. branching bacterium Aquifex pyrophilus. Syst. Appl. Microbiol. 16: 629- Albertano, P. and L. Kovacik. 1994. Is the genus LeptolynglYya (Cyano 637. phyte) a homogeneous taxon? Arch. Hydrobiol. Suppl. 105: 37-51. Achenbach-Richter, L., R Gupta, KO. Stetter and C.R Woese. 1987. Were Aldrich, H.C., D.B. Beimborn and P. Schönheit. 1987. Creation of arti the original eubacteria thermophiles? Syst. Appl. Microbiol. 9: 34- factual internal membranes during fixation of Methanobacterium ther 39. moautotrophicum. Can.]. Microbiol. 33: 844-849. Adams, D.G., D. Ashworth and B. -
Soil Microbiome Manipulation Gives New Insights in Plant Disease-Suppressive Soils from the Perspective of a Circular Economy: a Critical Review
sustainability Review Soil Microbiome Manipulation Gives New Insights in Plant Disease-Suppressive Soils from the Perspective of a Circular Economy: A Critical Review Ugo De Corato Department of Bioenergy, Biorefinery and Green Chemistry (TERIN-BBC-BIC), Italian National Agency for New Technologies, Energy and Sustainable Economic Development (ENEA), 70124 Bari, Italy; [email protected]; Tel.: +39-0831-201-616 Abstract: This review pays attention to the newest insights on the soil microbiome in plant disease- suppressive soil (DSS) for sustainable plant health management from the perspective of a circular economy that provides beneficial microbiota by recycling agro-wastes into the soil. In order to increase suppression of soil-borne plant pathogens, the main goal of this paper is to critically discuss and compare the potential use of reshaped soil microbiomes by assembling different agricultural practices such as crop selection; land use and conservative agriculture; crop rotation, diversifica- tion, intercropping and cover cropping; compost and chitosan application; and soil pre-fumigation combined with organic amendments and bio-organic fertilizers. This review is seen mostly as a comprehensive understanding of the main findings regarding DSS, starting from the oldest concepts to the newest challenges, based on the assumption that sustainability for soil quality and plant health is increasingly viable and supported by microbiome-assisted strategies based on the next-generation sequencing (NGS) methods that characterize in depth the soil bacterial and fungal communities. This approach, together with the virtuous reuse of agro-wastes to produce in situ green composts and organic bio-fertilizers, is the best way to design new sustainable cropping systems in a circular economy system.