Mass Balance Models.Pdf
Total Page:16
File Type:pdf, Size:1020Kb
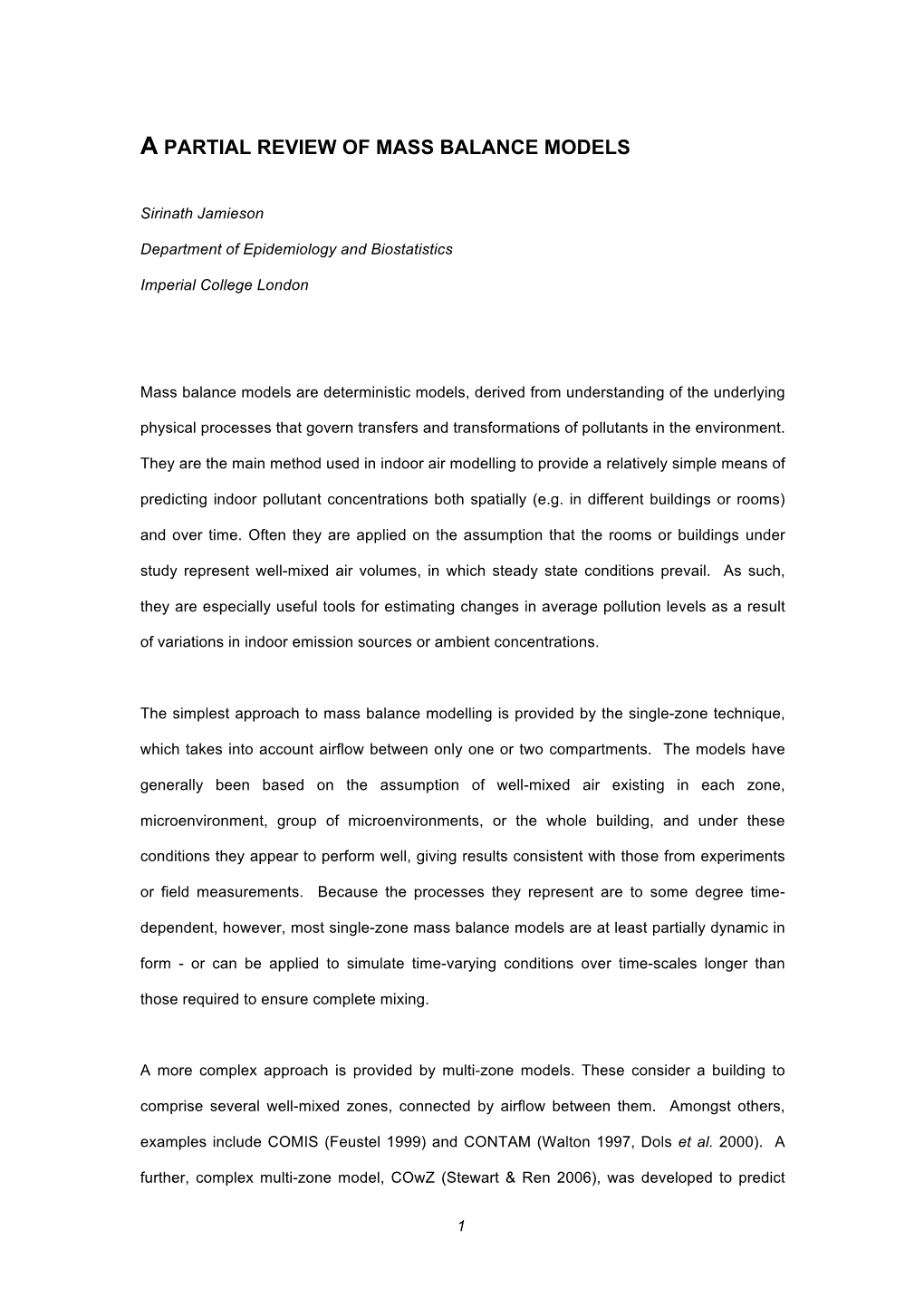
Load more
Recommended publications
-
On the Multi-Physics of Mass-Transfer Across Fluid Interfaces
On the multi-physics of mass-transfer across fluid interfaces Dieter Bothe Center of Smart Interfaces TU Darmstadt [email protected] Abstract Mass transfer of gaseous components from rising bubbles to the ambient liquid can be described based on continuum mechanical sharp-interface balances of mass, momentum and species mass. In this context, the stan- dard model consists of the two-phase Navier-Stokes equations for incom- pressible fluids with constant surface tension, complemented by reaction- advection-diffusion equations for all constituents, employing Fick’s law. This standard model is inconsistent with the continuity equation, the mo- mentum balance and the second law of thermodynamics. The present paper reports on the details of these severe shortcomings and provides thermodynamically consistent model extensions which are required to cap- ture various phenomena which occur due to the multi-physics of interfacial mass transfer. In particular, we provide a simple derivation of the interface Maxwell-Stefan equations which does not require a time scale separation, while the main contribution is to show how interface concentrations and interface chemical potentials mediate the influence on mass transfer of a transfer component exerted by the change in interface energy due to an adsorbing surfactant. Keywords: Soluble surfactant, interface chemical potentials, interfacial en- tropy production, interface Maxwell-Stefan equations, jump conditions, surface tension effects, high Schmidt number problem, artificial boundary conditions. Introduction The standard model for the continuum mechanical description of mass transfer across fluid interfaces is based on the incompressible two-phase Navier-Stokes equations for fluid systems without phase change. To be more precise, inside the fluid phases the governing equations are arXiv:1501.05610v1 [physics.flu-dyn] 22 Jan 2015 ∇ · v =0, (1) visc ∂t(ρv)+ ∇ · (ρv ⊗ v)+ ∇p = ∇ · S + ρg (2) with the viscous stress tensor T Svisc = η(∇v + ∇v ), (3) 1 where the material parameters depend on the respective phase. -
MATERIAL BALANCE NOTES Revision 3 Irven Rinard Department
MATERIAL BALANCE NOTES Revision 3 Irven Rinard Department of Chemical Engineering City College of CUNY and Project ECSEL October 1999 © 1999 Irven Rinard CONTENTS INTRODUCTION 1 A. Types of Material Balance Problems B. Historical Perspective I. CONSERVATION OF MASS 5 A. Control Volumes B. Holdup or Inventory C. Material Balance Basis D. Material Balances II. PROCESSES 13 A. The Concept of a Process B. Basic Processing Functions C. Unit Operations D. Modes of Process Operations III. PROCESS MATERIAL BALANCES 21 A. The Stream Summary B. Equipment Characterization IV. STEADY-STATE PROCESS MODELING 29 A. Linear Input-Output Models B. Rigorous Models V. STEADY-STATE MATERIAL BALANCE CALCULATIONS 33 A. Sequential Modular B. Simultaneous C. Design Specifications D. Optimization E. Ad Hoc Methods VI. RECYCLE STREAMS AND TEAR SETS 37 A. The Node Incidence Matrix B. Enumeration of Tear Sets VII. SOLUTION OF LINEAR MATERIAL BALANCE MODELS 45 A. Use of Linear Equation Solvers B. Reduction to the Tear Set Variables C. Design Specifications i VIII. SEQUENTIAL MODULAR SOLUTION OF NONLINEAR 53 MATERIAL BALANCE MODELS A. Convergence by Direct Iteration B. Convergence Acceleration C. The Method of Wegstein IX. MIXING AND BLENDING PROBLEMS 61 A. Mixing B. Blending X. PLANT DATA ANALYSIS AND RECONCILIATION 67 A. Plant Data B. Data Reconciliation XI. THE ELEMENTS OF DYNAMIC PROCESS MODELING 75 A. Conservation of Mass for Dynamic Systems B. Surge and Mixing Tanks C. Gas Holders XII. PROCESS SIMULATORS 87 A. Steady State B. Dynamic BILIOGRAPHY 89 APPENDICES A. Reaction Stoichiometry 91 B. Evaluation of Equipment Model Parameters 93 C. Complex Equipment Models 96 D. -
Introduction to Oxidation and Mass & Energy Balances
Introduction to Oxidation and Mass & Energy Balances Michael Mannuzza IT3/HWC OBG BaltimoreBaton Rouge,2014 LA 2016 Combustion • Combustion is an oxidation reaction: Fuel + O2 ---> Products of Combustion + Heat Energy • In addition to traditional burner fuels, incinerator fuel can include solid, gaseous and liquid waste. IT3/HWC • The Products of Combustion (POC) are the BaltimoreBaton primary concern from an Air Pollution Control Rouge,2014 LA 2016 (APC) perspective. Air Pollutants and Regulatory Drivers • The type of APC system required for an incinerator will be decided based on the system’s POCs and the regulatory limits mandated for specific pollutants. • Emissions of the following pollutants are typically regulated for most incinerator applications: • IT3/HWC • Dioxin/Furans NOx • Particulate Matter BaltimoreBaton • Mercury (Hg) Rouge,2014 LA • VOCs & Total Hydrocarbons 2016 • Semi-Volatile Metals (Cd, Pb) • Carbon Monoxide • Low-Volatility Metals (As, Be, Cr) • HCl & Cl2 • SOx • Others Identifying APC Requirements Three Steps: 1. Quantify anticipated POCs 2. Identify Regulatory Emission Constraints (establish abatement requirements) IT3/HWC 3. Quantify the discharge flow rate of the system. BaltimoreBaton Rouge,2014 LA 2016 Waste Feed Properties I. Begin by identifying the properties of the waste feed and quantifying the constituency of the waste: 1. Material Take-Offs 2. Proximate, Ultimate, and Ash Analysis 3. Sample & Analyze for Specific Compounds 4. Employ Other Empirical Methods PROXIMATE ANALYSIS ULTIMATE ANALYSIS ASH ANALYSIS -
1Eng -Design Basics, Mass Balance, Kinetics.Pdf
Applied chemical process Basic terms, mass balance Engineering thinking description of the industrial apparatus + description of the chemical and physical operation in the process precise formulation of the problem + design the right solution Process technology development design finish design development end of end construction of KH delivery KH Intensity Intensity year 1 Operations • Chemical processes - reactors • Mechanical processes - transport, mixing, filtration, grinding, sedimentation… • Diffusion processes - extraction, distillation, crystallization, drying • Thermal processes – coolig, warming-up, condensation, evaporation Description of the technology Maximal yield x minimum cost • Amount and composition of the products – reactants, products, waste (material balance) • Energy consumption – stream, cooling water, electrical energy, cooling air (enthalpy balance) • Industrial devices – wattage, type, dimension, output (design and check calculation) • Costs – raw materials, energy, investments, payroll (economic balance) Economic efficiency of the process • Costing and price of the product • Energy costs • Depreciation and maintenance • Cost of human work = Production costs + Corporate overhead = Complete costs + Profit = Total Price 2 Cost versus production - development of a new product 1) Old process production fall off Not simultaneously 2) Application of the modern technology Influence of capacity to the relative cost raw materials cost relative invest cost energy capacity (t/year) Type of the system – based on the exchange -
Applicability of Simple Mass and Energy Balances in Food Drum Drying
J. Basic. Appl. Sci. Res., 4(1)128-133, 2014 ISSN 2090-4304 © 2014, TextRoad Publication Journal of Basic and Applied Scientific Research www.textroad.com Applicability of Simple Mass and Energy Balances in Food Drum Drying Tomislav Jurendić Bioquanta Ltd, Dravska 17, 48000 Koprivnica, Croatia Received: November 16 2013 Accepted: December 10 2013 ABSTRACT Drum drying is one of the most important drying methods in the production of dried food. Drum dryers are robust in construction and consume huge quantity of energy. Holding the drying process under control can be of high importance for both saving the energy and reducing the costs. Mass and energy balances represent a very good basis towards efficient process control and quick estimation of the process state, as well as are unavoidable step in the design of food processes, respectively. In this work the using of simple mass and energy balances in practical industrial applications is demonstrated. KEY WORDS: baby food, drum drying, energy balance, mass balance, process calculations 1. INTRODUCTION Drum drying (Figure 1) has been widely used in food industry for drying of various liquid, semiliquid and paste-like food materials. That way, many dried food products such as milk powder, baby foods, starch, fruit and vegetables pulps, honey, malto-dextrins, yeast creams and many other food and non-food products can be produced [1-6]. The obtained dried product is porous and easy to rehydrate, ready to use [6]. As well, the manufactured dried products can be employed as semi-finished products in milk, drinking, confectionary and other industries [4]. However, the purpose of food drying is to extend shelf life of products with minimum packaging requirements and reduced shipping weights [4]. -
Glossary of Glacier Mass Balance and Related Terms
The University of Manchester Research Glossary of glacier mass balance and related terms Link to publication record in Manchester Research Explorer Citation for published version (APA): Cogley, J. G., Arendt, A. A., Bauder, A., Braithwaite, R. J., Hock, R., Jansson, P., Kaser, G., Moller, M., Nicholson, L., Rasmussen, L. A., & Zemp, M. (2010). Glossary of glacier mass balance and related terms. (IHP-VII Technical Documents in Hydrology). International Hydrological Programme. Citing this paper Please note that where the full-text provided on Manchester Research Explorer is the Author Accepted Manuscript or Proof version this may differ from the final Published version. If citing, it is advised that you check and use the publisher's definitive version. General rights Copyright and moral rights for the publications made accessible in the Research Explorer are retained by the authors and/or other copyright owners and it is a condition of accessing publications that users recognise and abide by the legal requirements associated with these rights. Takedown policy If you believe that this document breaches copyright please refer to the University of Manchester’s Takedown Procedures [http://man.ac.uk/04Y6Bo] or contact [email protected] providing relevant details, so we can investigate your claim. Download date:09. Oct. 2021 GLOSSARY OF GLACIER MASS BALANCE AND RELATED TERMS Prepared by the Working Group on Mass-balance Terminology and Methods of the International Association of Cryospheric Sciences (IACS) IHP-VII Technical Documents in Hydrology No. 86 IACS Contribution No. 2 UNESCO, Paris, 2011 Published in 2011 by the International Hydrological Programme (IHP) of the United Nations Educational, Scientific and Cultural Organization (UNESCO) 1 rue Miollis, 75732 Paris Cedex 15, France IHP-VII Technical Documents in Hydrology No. -
Entropy Production As a Measure for Resource Use
Entropy production as a measure for resource use Method development and application to metallurgical processes D I S S E R T A T I O N zur Erlangung des Doktorgrades des Fachbereichs Physik der Universit¨at Hamburg vorgelegt von Stefan G¨oßling aus Herford Hamburg 2001 Gutachter der Dissertation: Prof. Dr. H. Spitzer Prof. Dr. G. Mack Gutachter der Disputation: Prof. Dr. H. Spitzer Prof. Dr. G. Zimmerer Datum der Disputation: 23. 10. 2001 Sprecher des Fachbereichs Physik und Vorsitzender des Promotionsausschusses Prof. Dr. F.{W. Bußer¨ There are only two things in the world that grow when you give them away: Love and Entropy (Anonymous) Abstract In this thesis, entropy production is introduced as a measure for resource use based on the laws of thermodynamics. The main motivation for the development of this measure is given by two facts: resource use is an important parameter in the ecological assessment of human activity and there exists as yet no satisfactory measure for the resource use of industrial processes. Also, linking ecological and economical aspects of industrial processes, entropy production and entropy export play an important role for the development of living structures. On the grounds of the laws of thermodynamics, the mathematical framework for analysing the entropy production of arbitrary processes is developed, including a method to compensate for incomplete data. Several basic applications of the concept of entropy analysis are given, which verify the proposition that entropy production and resource use are equivalent. In order to prove the method's practicability, an industrial-scale case-study is performed on the production of copper from ore concentrates and secondary (recycled) material. -
Introduction to Combustion Analysis
College of Engineering and Computer Science Mechanical Engineering Department Mechanical Engineering 483 Alternative Energy Engineering II Spring 2010 Number: 17724 Instructor: Larry Caretto Introduction to Combustion Analysis INTRODUCTION These notes introduce simple combustion models. Such models can be used to analyze many industrial combustion processes and determine such factors as energy release and combustion efficiency. They can also be used to relate measured pollutant concentrations to other measures of emissions such as mole fractions, mass fractions, mass of pollutant per unit mass of fuel, mass of pollutant per unit fuel heat input, etc. These notes provide the derivation of all the equations that are used for these relations. BASIS OF THE ANALYSIS The simple combustion model assumes complete combustion in which all fuel carbon forms CO2, all fuel hydrogen forms H2O, all fuel sulfur forms SO2, and all fuel nitrogen forms N2. This provides results that are accurate to about 0.1% to 0.2% for well operating combustion processes in stationary combustion devices. It is not valid when the combustion process is fuel rich. Complete combustion of model fuel formula with oxygen – The typical fuel can be represented by the fuel formula CxHySzOwNv. With this fuel formula we can analyze a variety of fuels including pure chemical compounds, mixtures of compounds, and complex fuels such as petroleum and coals where the elemental composition of the fuel is measured. This type of measurement is said to provide an ultimate analysis. Regardless of the source of data for x, y, z, w and v, the mass of fuel represented by this formula1 can be found from the atomic weights of the various elements: M fuel = x M C + y M H + z M S + w M O + v M N [1] The oxygen required for an assumed complete combustion of this fuel formula can is determined from the oxygen requirements for complete combustion of the individual elements. -
Chemical Engineering Kinetics
CHEMICAL ENGINEERING KINETICS Based on CHEM_ENG 408 at Northwestern University BRIEF REVIEW OF REACTOR ARCHETYPES | 1 TABLE OF CONTENTS 1 Brief Review of Reactor Archetypes .................................................................................... 3 1.1 The Mass Balance ......................................................................................................................... 3 1.2 Batch Reactor ................................................................................................................................ 3 1.3 Continuous-Stirred Tank Reactor ................................................................................................. 4 1.4 Plug-Flow Reactor ........................................................................................................................ 4 2 Power Law Basics .................................................................................................................. 6 2.1 Arrhenius Equation ....................................................................................................................... 6 2.2 Mass-Action Kinetics .................................................................................................................... 7 2.2.1 Overview ................................................................................................................................................. 7 2.2.2 First Order Kinetics ................................................................................................................................ -
Principles of Momentum, Mass and Energy Balances - Leon Gradoń
CHEMICAL ENGINEEERING AND CHEMICAL PROCESS TECHNOLOGY – Vol. I -Principles of Momentum, Mass and Energy Balances - Leon Gradoń PRINCIPLES OF MOMENTUM, MASS AND ENERGY BALANCES Leon Gradoń Faculty of Chemical and Process Engineering, Warsaw University of Technology, Warsaw, Poland Keywords: Steady-state, nonsteady-state, continuous, batch, differential balance, integral balance, accumulation, stress, tensor, residence time, intensity function, birth function, death function Contents 1. Introduction 2. Macroscopic balances 2.1. Process Classification and Types of Balances 2.2. Mass Balances 2.3. Energy Balances 3. Microscopic balances 3.1. Continuum and Field Quantities 3.2. Conservation Equation for Continuum 3.3. Balance of Linear Momentum 3.4. Mass Balance 3.5. Energy Balance 4.1. Age Distribution Functions 4.2. General Population Balance 4. Population balances Glossary Bibliography Biographical Sketch Summary Balance of the entity producing accumulation is, particularly, a basic source of quantitative models of phenomena or processes. The concept of balance of momentum, mass, and energyUNESCO defined in the chapter is– used EOLSS for elaboration of the algebraic or differential equation which describes processes at the macroscopic or microscopic levels of observation. The procedure of macroscopic balancing for continuous and batch processes is presented. Differential balance equations are formulated for momentum, mass, and energySAMPLE through the contribution ofCHAPTERS local rates of transport expressed by principal Newton’s, Fick’s and Fourier’s laws. For description of more complex systems in which strong turbulence of the fluid flow and/or vessel geometry are involved and characterization of the product property is necessary, the population balances are required. Concepts of the age distribution function and the intensity function are introduced and incorporated into the general population balance simultaneously with function of birth and death of balanced entity. -
CHAPTER 2 MATERIAL and ENERGY BALANCES Material
CHAPTER 2 MATERIAL AND ENERGY BALANCES Material quantities, as they pass through food processing operations, can be described by material balances. Such balances are statements on the conservation of mass. Similarly, energy quantities can be described by energy balances, which are statements on the conservation of energy. If there is no accumulation, what goes into a process must come out. This is true for batch operation. It is equally true for continuous operation over any chosen time interval. Material and energy balances are very important in the food industry. Material balances are fundamental to the control of processing, particularly in the control of yields of the products. The first material balances are determined in the exploratory stages of a new process, improved during pilot plant experiments when the process is being planned and tested, checked out when the plant is commissioned and then refined and maintained as a control instrument as production continues. When any changes occur in the process, the material balances need to be determined again. The increasing cost of energy has caused the food industry to examine means of reducing energy consumption in processing. Energy balances are used in the examination of the various stages of a process, over the whole process and even extending over the total food production system from the farm to the consumer’s plate. Material and energy balances can be simple, at times they can be very complicated, but the basic approach is general. Experience in working with the simpler systems such as individual unit operations will develop the facility to extend the methods to the more complicated situations, which do arise. -
Chapter 4 Mass and Energy Balances
Chapter 4 Mass and Energy Balances In this chapter we will apply the conservation of mass and conservation of energy laws to open systems or control volumes of interest. The balances will be applied to steady and unsteady system such as tanks, turbines, pumps, and compressors. 4.1 Conservation of Mass The general balance equation can be written as Accumulation = Input + Generation - Output - Consumption The terms (Generation - Consumption) are usually combined to call Generation with positive value for net generation and negative value for net consumption. Let mcv = total mass (kg) of A within the system at any time. m" in = rate (kg/s) at which A enters the system by crossing the boundaries. m" out = rate (kg/s) at which A leaves the system by crossing the boundaries. r"gen = rate (kg/s) of generation of A within the system by chemical reactions. r"cons = rate (kg/s) of consumption of A within the system by chemical reactions. Then the mass balance on species A can be written as dm cv = m" + r" − m" − r" (4.1-1) dt in gen out cons If there is no chemical reaction, the mass balance equation is simplified to dm cv = m" − m" (4.1-2) dt in out Example 4.1-1 ---------------------------------------------------------------------------------- Balance on an interest earning checking account during month of February Withdrawn = $525.00 Deposit = $1000.00 Interest = $3.00 Fees = $5.00 Solution ------------------------------------------------------------------------------------------ Accumulation = $1000 + $3 - $525 - $5 = $477.00 /month (February) 4-1 Example 4.1-2 ---------------------------------------------------------------------------------- Snake-Eyes Maggoo is a man of habit. For instance, his Friday evenings are all alike-into the joint with his week’s salary of $180, steady gambling at “2-up” for two hours, then home to his family leaving $45 behind.