High Microfilament Concentration Results in Barbed-End ADP Caps
Total Page:16
File Type:pdf, Size:1020Kb
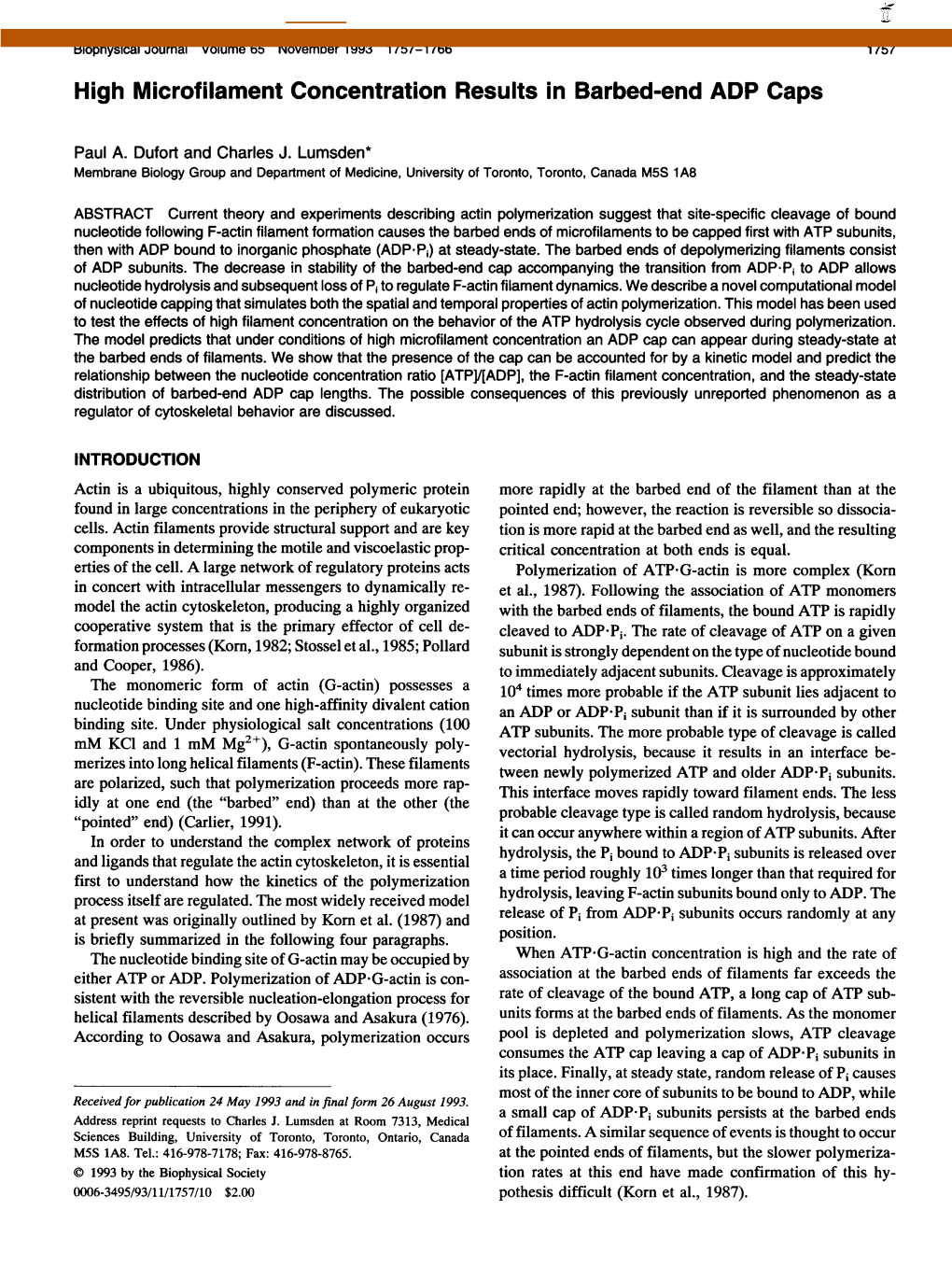
Load more
Recommended publications
-
ATP and Cellular Work | Principles of Biology from Nature Education
contents Principles of Biology 23 ATP and Cellular Work ATP provides the energy that powers cells. Magnetic resonance images of three different areas in the rat brain show blood flow and the biochemical measurements of ATP, pH, and glucose, which are all measures of energy use and production in brain tissue. The image is color-coded to show spatial differences in the concentration of these energy-related variables in brain tissue. © 1997 Nature Publishing Group Hoehn-Berlage, M., et al. Inhibition of nonselective cation channels reduces focal ischemic injury of rat brain. Journal of Cerebral Blood Flow and Metabolism 17, 534–542 (1997) doi: 10.1097/00004647-199705000-00007. Used with permission. Topics Covered in this Module Using Energy Resources For Work ATP-Driven Work Major Objectives of this Module Describe the role of ATP in energy-coupling reactions. Explain how ATP hydrolysis performs cellular work. Recognize chemical reactions that require ATP hydrolysis. page 116 of 989 4 pages left in this module contents Principles of Biology 23 ATP and Cellular Work Energy is a fundamental necessity for all of life's processes. Without energy, flagella cannot move, DNA cannot be unwound or separated for replication or gene expression, cells cannot divide, plants cannot grow and animals cannot reproduce. Energy is vital, but where does it come from? Plants and photosynthetic microbes capture light energy and convert it into chemical energy for their own use. Organisms that cannot produce their own food, such as fungi and animals, feed upon this captured energy. However, the chemical energy produced by photosynthesizers needs to be converted into a usable form. -
Structural Insight Into Signal Conversion and Inactivation by Ntpdase2 in Purinergic Signaling
Structural insight into signal conversion and inactivation by NTPDase2 in purinergic signaling Matthias Zebisch and Norbert Stra¨ ter* Center for Biotechnology and Biomedicine, Institute of Bioanalytical Chemistry, Faculty of Chemistry and Mineralogy, University of Leipzig, Deutscher Platz 5, 04103 Leipzig, Germany Communicated by William N. Lipscomb, Harvard University, Cambridge, MA, March 13, 2008 (received for review January 4, 2008) Cell surface-located nucleoside triphosphate diphosphohydrolases prominent DXG motif and correspond to the - and ␥-phos- (NTPDase1, -2, -3, and -8) are oligomeric integral membrane pro- phate binding loops of soluble members of the actin/hsp70/sugar teins responsible for signal conversion and inactivation in extra- kinase superfamily (17). cellular nucleotide-mediated ‘‘purinergic’’ signaling. They catalyze NTPDases differ significantly in product formation. This is of the sequential hydrolysis of the signaling molecule ATP via ADP to considerable relevance for the regulation of nucleotide signaling. AMP. Here we present the structure of the extracellular domain of NTPDase1, which corresponds to the lymphoid cell activation Rattus norvegicus NTPDase2 in an active state at resolutions antigen CD39, hydrolyzes ATP processively to AMP [i.e., without between 1.7 Å and 2.1 Å in four different forms: (i) apo form, (ii) release of the intermediate ADP from the active site (18, 19)]. The ternary complex with the nonhydrolyzable ATP analog AMPPNP ectoenzyme is expressed by cells of the immune system, quiescent and cofactor Ca2؉,(iii) quaternary complex with Ca2؉ and bound endothelial cells, vascular smooth muscle cells, and others. Because products AMP and phosphate, and (iv) binary product complex of its ability to hydrolyze both ATP and the platelet activator ADP with AMP only. -
The Hydrolysis of ATP and Related Nucleotides by Ehrlich Ascites Carcinoma Cells*
The Hydrolysis of ATP and Related Nucleotides by Ehrlich Ascites Carcinoma Cells* DONALD F. HOELZL WALLACH AND DONNA ULLREY (Department of Biological Chemistry, Harvard Medical School, and Protein Foundation, Boston, Mass.) SUMMARY The ATPase of Ehrlich ascites carcinoma cells has been examined. The evidence presented indicates the following: (a) ATP hydrolysis occurs at the cell surface, (b) the cell membrane goes into the microsomal fraction upon cell rupture and differ ential centrifugation, (c) ATP hydrolysis depends upon the nature and concentration of external activator ions, (d) hydrolysis is not limited to ATP; other nucleoside tn phosphates are attacked with equal vigor, and ADP and AMP are also hydrolyzed, (e) ATP hydrolysis appears to proceed in stepwise fashion via ADP and AMP to ade nine and inorganic phosphorus, (J) ATP, but not ADP or ITP hydrolysis, is stimulated by 92,4-dinitrophenol, but this effect is reversed by glucose. The presence on the surface of a variety of phate by Ehrlich ascites carcinoma cells was first cells of enzymes capable of hydrolyzing adenosine recorded by Acs et at. (92). triphosphate has been reported by several workers. The above studies suggest the possibility that Rothstein et al have presented evidence for the adenosine tniphosphate-hydrolyzing enzymes may localization of an adenosine tniphosphatase on the exist as an integral part of the lipoprotein matrix surface of yeast (920) and of intestinal epithelium of cell membranes. In the course of examining (921). Libet (13) and Abood et a! (1) have shown this hypothesis for the case of the Ehrlich ascites that the adenosine triphosphatase of the giant carcinoma, we have studied in some detail the axon of the squid lies in the axon sheath rather cleavage of adenosine triphosphate and related than in the axoplasm. -
The Connection Between Actin Atpase and Polymerization
Chapter 3 The Connection Between Actin ATPase and Polymerization Herwig Schu¨ler,1 Roger Karlsson,2 Clarence E. Schutt,3 and Uno Lindberg2 1Max Delbrueck Center for Molecular Medicine Neuroproteomics, D‐13125, Berlin‐Buch, Germany 2Department of Cell Biology, The Wenner‐Gren Institute, Stockholm University, SE‐106 91 Stockholm, Sweden 3Department of Chemistry, Princeton University, Princeton, New Jersey 08544 I. Actin Microfilament System II. Atomic Structure of the Actin Monomer III. Profilin: ‐Actin Crystal IV. Interdomain Connectivity in Actin V. Actin ATPASE A. Monomeric Actin Hydrolyzes ATP B. Polymer Formation and ATP Hydrolysis C. The Actin–ATP/ADP⋅Pi Cap VI. Mechanism of ATP Hydrolysis on Actin A. Active Site Nucleophile B. Catalytic Base(s) VII. Actin Methylhistidine 73, ATPase, Phosphate Release, and Polymerization VIII. Importance of the Status of the Actin‐Bound Nucleotide References Remodeling of the actin filament system in cells results from strictly regulated polymerization and depolymerization of actin, where hydrolysis of actin‐bound ATP is crucial. Actin–actin interactions are influenced by the state of the bound nucleotide, and many microfilament regulators influence the actin ATPase by binding preferen- tially either to ATP/ADPÁPi‐ or ADP‐bound actin. This chapter summarizes observa- tions made concerning the actin ATPase and its role in the biological activity of actin and actin filaments. I. ACTIN MICROFILAMENT SYSTEM Actin and myosin, organized into supramolecular structures, cooperate to generate the force necessary for many types of dynamic cellular transport processes. As part of the energy‐transducing mechanism in muscle cells, they generate large‐scale movements. Advances in Molecular and Cell Biology, Vol. -
ATP-Driven Active Transport in Right-Side-Out Bacterial Membrane
Proc. NatL Acad. Sci. USA Vol. 78, No. 6, pp. 3446-3449, June 1981 Biochemistry ATP-driven active transport in right-side-out bacterial membrane vesicles (Salmonella typhimurium/Escherichia coli/phosphoglycerate transport/electrochemical proton gradient/cloning) JEROEN HUGENHOLTZ*, JEN-SHIANG HONGt, AND H. RONALD KABACK*t *lAboratory of Membrane Biochemistry, Roche Institute of Molecular Biology, Nutley, New Jersey 07110; and tGraduate Department of Biochemistry, Brandeis University, Waltham, Massachusetts 02154 Communicated by Sidney Udenfrtend, March 2, 1981 ABSTRACT Membrane vesicles from Salmonella typhimurium phimurium LT-2 that catalyzes the uptake of2-phosphoglycer- induced for phosphoglycerate transport, were loaded with pyru- ate, 3-phosphoglycerate, and phosphoenolpyruvate, and, sub- vate kinase and ADP by lysing spheroplasts under appropriate sequently, they demonstrated that the transport system allows conditions. Vesicles so prepared catalyze active transport of pro- energy-depleted cells to use external phosphoenolpyruvate line and serine in the presence of phosphoenolpyruvate; this ac- more efficiently for vectorial phosphorylation of methyl a-D- tivity is abolished by the protonophore carbonyl cyanide-m-chlo- glucopyranoside (33). These workers also suggested the possi- rophenylhydrazone and by the HW-ATPase inhibitor NN' bility of using the phosphoglycerate transport system to affect dicyclohexylcarbodiimide but not by anoxia or cyanide. In con- the intravesicular generation ofATP from phosphoenolpyruvate trast, D-lactate-driven -
Hydrolysis of Extracellular ATP by Vascular Smooth Muscle Cells Transdifferentiated Into Chondrocytes Generates Pi but Not Ppi
International Journal of Molecular Sciences Article Hydrolysis of Extracellular ATP by Vascular Smooth Muscle Cells Transdifferentiated into Chondrocytes Generates Pi but Not PPi Rene Buchet 1,* , Camille Tribes 1, Valentine Rouaix 1, Bastien Doumèche 1 , Michele Fiore 1 , Yuqing Wu 2, David Magne 1 and Saida Mebarek 1 1 Institute for Molecular and Supramolecular Chemistry and Biochemistry, Université Lyon 1, French National Centre for Scientific Research, F-69622 Lyon, France; [email protected] (C.T.); [email protected] (V.R.); [email protected] (B.D.); michele.fi[email protected] (M.F.); [email protected] (D.M.); [email protected] (S.M.) 2 State Key Laboratory of Supramolecular Structure and Materials, Institute of Theoretical Chemistry, Jilin University, Changchun 130012, China; [email protected] * Correspondence: [email protected] Abstract: (1) Background: Tissue non-specific alkaline phosphatase (TNAP) is suspected to induce atherosclerosis plaque calcification. TNAP, during physiological mineralization, hydrolyzes the min- eralization inhibitor inorganic pyrophosphate (PPi). Since atherosclerosis plaques are characterized by the presence of necrotic cells that probably release supraphysiological concentrations of ATP, we explored whether this extracellular adenosine triphosphate (ATP) is hydrolyzed into the mineraliza- Citation: Buchet, R.; Tribes, C.; tion inhibitor PPi or the mineralization stimulator inorganic phosphate (Pi), and whether TNAP is Rouaix, V.; Doumèche, B.; Fiore, M.; involved. (2) Methods: Murine aortic smooth muscle cell line (MOVAS cells) were transdifferentiated Wu, Y.; Magne, D.; Mebarek, S. into chondrocyte-like cells in calcifying medium, containing ascorbic acid and β-glycerophosphate. Hydrolysis of Extracellular ATP by ATP hydrolysis rates were determined in extracellular medium extracted from MOVAS cultures Vascular Smooth Muscle Cells during their transdifferentiation, using 31P-NMR and IR spectroscopy. -
30 the Technical Assistance of My Wife, Mrs. I. SJ6QUIST and of Miss A
30 j. SJ(')QUIST ACKNOWLEDGEMENTS The technical assistance of my wife, Mrs. I. SJ6QUIST and of Miss A. WELIN is gratefully acknowledged. This work was supported by grants from "Statens Medicinska ForskningsrSd". REFERENCES 1 p. EDMAN, .4cta Chem. Scan&, 4 (~95 °) 277. 2 p. EDMAN, Acta Chem. Scand., 4 (195 ° ) 283. 3 j. Sj6QUIST, Acla Chem. Scand., 7 (1953) 447. 4 ~p. EDMAN AND J. SJ6QUIST, Acta Chem. Scand., lo (1956) 15o7. 5 j. Sj6QUIST, Biochim. Biophys. ~4cta, 16 (1955) 283. 6 j. SjoQUIST, .drkiv Kemi, ii (I957) ~29, 15l. 7 C. I-[. \V. HIRS, J. Biol. Chenz., 219 (1956) 611. 8 A. L. LEVY AND D. CItUNG, Biochim. Biophys. Acta, 17 (1955) 454. 9 }V. H, STEIN AND S. MOORE, J. Biol. Chem., l 78 (1949) 79. 10 M. V~~. REES, Biochem. dr., 4 ° (1946) 632. n C. I~. \¥. HIRS, \V. N. STEIN AND S. MOORt~, J. Biol. Chem., 211 (1954) 94 I. Biochim. Biophys. Acla, 41 (196o) 20-30 HYDROLYSIS OF NUCLEOSIDE DI-AND TRIPHOSPHATES BY CRYSTALLINE PREPARATIONS OF YEAST INORGANIC PYROPHOSPHATASE MILTON J. SCHLESINGER* AND MINOR J. COON Department o t Biological Chemistry, Medical School, The University o/Michigc~n, Hnn Arbor, Mich. (U.S.A.) (Received October 3ist, 1959) SUMMARY I. Crystalline preparations of yeast inorganic pyrophosphatase catalyze phos- phate liberation from ATP and ADP, as well as from pyrophosphate, in the presence of zinc ions. 2. A variety of other nucleoside di- and triphosphates are hydrolyzed in the presence of zinc ions and pyrophosphatase, and manganous and cobaltous ions can partially substitute for zinc in effecting ATP hydrolysis. -
The Actin-Myosin Interaction in Muscle: Background and Overview
International Journal of Molecular Sciences Editorial Special Issue: The Actin-Myosin Interaction in Muscle: Background and Overview John Squire 1,2 1 Muscle Contraction Group, School of Physiology, Pharmacology and Neuroscience, University of Bristol, Bristol BS8 1TD, UK; [email protected]; Tel.: +44-7706-07-6383 2 Department of Surgery and Cancer, Faculty of Medicine, Imperial College London, London SW7 2BZ, UK Received: 11 October 2019; Accepted: 15 October 2019; Published: 14 November 2019 Abstract: Muscular contraction is a fundamental phenomenon in all animals; without it life as we know it would be impossible. The basic mechanism in muscle, including heart muscle, involves the interaction of the protein filaments myosin and actin. Motility in all cells is also partly based on similar interactions of actin filaments with non-muscle myosins. Early studies of muscle contraction have informed later studies of these cellular actin-myosin systems. In muscles, projections on the myosin filaments, the so-called myosin heads or cross-bridges, interact with the nearby actin filaments and, in a mechanism powered by ATP-hydrolysis, they move the actin filaments past them in a kind of cyclic rowing action to produce the macroscopic muscular movements of which we are all aware. In this special issue the papers and reviews address different aspects of the actin-myosin interaction in muscle as studied by a plethora of complementary techniques. The present overview provides a brief and elementary introduction to muscle structure and function and the techniques used to study it. It goes on to give more detailed descriptions of what is known about muscle components and the cross-bridge cycle using structural biology techniques, particularly protein crystallography, electron microscopy and X-ray diffraction. -
Mechanism of Na+-K+ Atpase Is As Follows
Input Template for Content Writers (e-Text and Learn More) 1. Details of Module and its Structure Module Detail Subject Name Botany Paper Name Cell Biology Module Name/Title Cell membrane and Cellular transport III Module Id <Module Id> Pre-requisites Objectives To understand principle and mechanism of primary active transport Keywords Active transport, Electochemical gradient Primary active transport, Facilitated Diffusion, Carrier proteins for primary active Structure of Module / Syllabus of a module (Define Topic / Sub-topic of module ) Passive transport <Definition>, <Types>, <Mechanism> Passive transport < Introduction > < Active transport>, < Electochemical gradient> < primary active transport> < Facilitated Diffusion > < carrier proteins for primary active transport > Management of Library and Information Network Library Science Network 2. 2. Development Team Role Name Affiliation National Coordinator <National Coordinator Name> Subject Coordinator Dr Sujata Bhargava Paper Coordinator Dr Nutan Malpathak Content Writer/Author (CW) Dr Pradnya Kedari Content Reviewer (CR) <CR Name> Language Editor (LE) <LE Name> Management of Library and Information Network Library Science Network TABLE OF CONTENTS (for textual content) 1 Introduction 2 Active transport 3 Electrochemical gradient 4 Primary active transport 5 Carrier Proteins or pumps for Active Transport Management of Library and Information Network Library Science Network Cell membrane and cellular transport III 1. Introduction Active transport is a transport of molecules against its concentration gradient. Molecules are carried from region of lower concentration to region of higher concentration. This process face resistance and so it has to utilise energy to overcome this resistance and carryout this transport. Therefore it is called as active transport. The process is also known as uphill transport. -
Lecture 1- Metabolism: Basic Concepts and Design 1 Introduction
Lecture 1- Metabolism: Basic Concepts and Design Chem 454: Regulatory Mechanisms in Biochemistry University of Wisconsin-Eau Claire 1 Introduction Questions we will focus on this semester: How does a cell extract energy and reducing power from its environment? How does a cell synthesize the building blocks of its macromolecules and the then the macromolecules themselves? How are these processes integrated and regulated? 2 2 Introduction Living organisms require an input of free energy to meet various needs: For mechanical work For active transport of molecules and ions For synthesis of biomolecules 3 3 Introduction Living organisms require an input of free energy Phototrophs Use energy from the sun to convert energy-poor molecules into energy rich molecules Chemotrophs Obtain energy by oxidizing the energy-rich molecules made by the phototrophs 4 4 Introduction Reduced molecules are energy-rich Oxidized molecules are energy-poor 5 5 Introduction Energy from photosynthesis or the oxidation of fuels can be transformed into an unequal distribution of ions across a biological membrane. The ion gradient can be used for Oxidative phosphorylation to make ATP Active transport across membranes Nerve transmission 6 6 Introductions Ion gradients: See Chapter 2.3.3 7 7 Metabolism Metabolism is composed of many coupled interconnecting reactions 8 8 Metabolism 9 9 Metabolism Classes of metabolic pathways: Catabolic pathways Those that convert energy into biologically useful forms Fuels (carbohydrates, fats) CO2 + H2O + useful energy Anabolic pathways Those that require an input of energy Useful energy + small molecules complex molecules 10 10 Metabolism Basic concepts of metabolism include: Thermodynamically unfavorable reactions can be driven by favorable reactions. -
ATP Analogues for Structural Investigations: Case Studies of a Dnab Helicase and an ABC Transporter
molecules Review ATP Analogues for Structural Investigations: Case Studies of a DnaB Helicase and an ABC Transporter 1,2, 1, , 1 3 Denis Lacabanne y, Thomas Wiegand * y , Nino Wili , Maria I. Kozlova , Riccardo Cadalbert 1, Daniel Klose 1, Armen Y. Mulkidjanian 3,4, Beat H. Meier 1,* and Anja Böckmann 5,* 1 Laboratory of Physical Chemistry, ETH Zurich, 8093 Zurich, Switzerland; [email protected] (D.L.); [email protected] (N.W.); [email protected] (R.C.); [email protected] (D.K.) 2 Medical Research Council Mitochondrial Biology Unit University of Cambridge, Cambridge Biomedical Campus, Keith Peters Building, Hills Road, Cambridge CB2 0XY, UK 3 Department of Physics, Osnabrueck University, 49069 Osnabrueck, Germany; [email protected] (M.I.K.); [email protected] (A.Y.M.) 4 School of Bioengineering and Bioinformatics and Belozersky Institute of Physico-Chemical Biology, Lomonosov Moscow State University, 119234 Moscow, Russia 5 Molecular Microbiology and Structural Biochemistry UMR 5086 CNRS/Université de Lyon, Labex Ecofect, 69367 Lyon, France * Correspondence: [email protected] (T.W.); [email protected] (B.H.M.); [email protected] (A.B.) These authors contributed equally to this work. y Academic Editor: Marilisa Leone Received: 17 October 2020; Accepted: 9 November 2020; Published: 12 November 2020 Abstract: Nucleoside triphosphates (NTPs) are used as chemical energy source in a variety of cell systems. Structural snapshots along the NTP hydrolysis reaction coordinate are typically obtained by adding stable, nonhydrolyzable adenosine triphosphate (ATP) -analogues to the proteins, with the goal to arrest a state that mimics as closely as possible a physiologically relevant state, e.g., the pre-hydrolytic, transition and post-hydrolytic states. -
Flagella-Driven Motility of Bacteria
biomolecules Review Flagella-Driven Motility of Bacteria Shuichi Nakamura 1 and Tohru Minamino 2,* 1 Department of Applied Physics, Graduate School of Engineering, Tohoku University, 6-6-05 Aoba, Aoba-ku, Sendai 980-8579, Japan 2 Graduate School of Frontier Biosciences, Osaka University, 1-3 Yamadaoka, Suita, Osaka 565-0871, Japan * Correspondence: [email protected]; Tel.: +81-6-6879-4625 Received: 27 June 2019; Accepted: 12 July 2019; Published: 14 July 2019 Abstract: The bacterial flagellum is a helical filamentous organelle responsible for motility. In bacterial species possessing flagella at the cell exterior, the long helical flagellar filament acts as a molecular screw to generate thrust. Meanwhile, the flagella of spirochetes reside within the periplasmic space and not only act as a cytoskeleton to determine the helicity of the cell body, but also rotate or undulate the helical cell body for propulsion. Despite structural diversity of the flagella among bacterial species, flagellated bacteria share a common rotary nanomachine, namely the flagellar motor, which is located at the base of the filament. The flagellar motor is composed of a rotor ring complex and multiple transmembrane stator units and converts the ion flux through an ion channel of each stator unit into the mechanical work required for motor rotation. Intracellular chemotactic signaling pathways regulate the direction of flagella-driven motility in response to changes in the environments, allowing bacteria to migrate towards more desirable environments for their survival. Recent experimental and theoretical studies have been deepening our understanding of the molecular mechanisms of the flagellar motor. In this review article, we describe the current understanding of the structure and dynamics of the bacterial flagellum.