Ellipticity, PDI and FLU, Evaluation of Aromaticity Indexes During Substituting B &N Atoms in Poly- Annulene
Total Page:16
File Type:pdf, Size:1020Kb
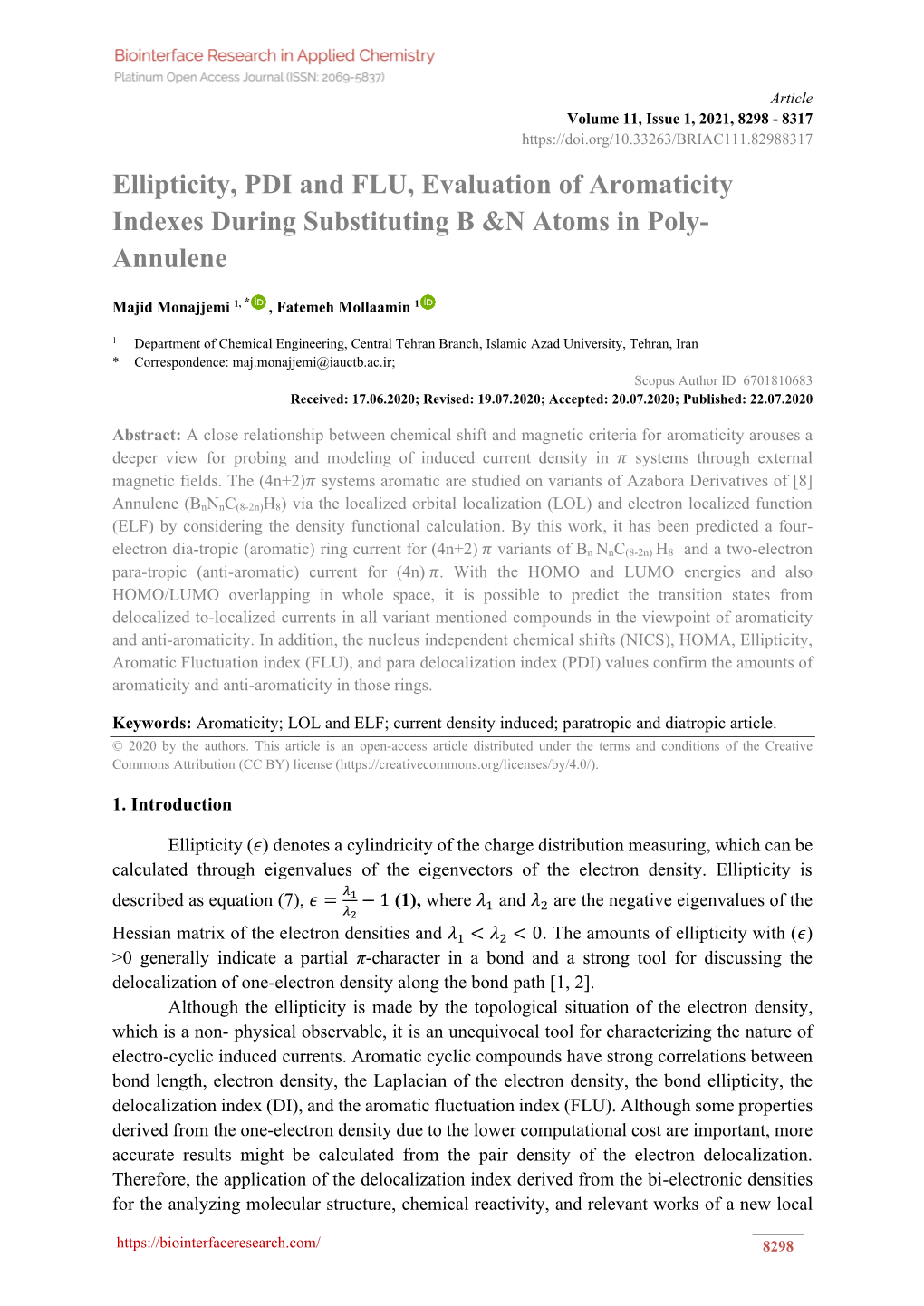
Load more
Recommended publications
-
On the Use of Energy Decomposition Analyses to Unravel the Origin of the Relative Stabilities of Isomers
ON THE USE OF ENERGY DECOMPOSITION ANALYSES TO UNRAVEL THE ORIGIN OF THE RELATIVE STABILITIES OF ISOMERS Majid El Hamdi Lahfid Dipòsit legal: Gi. 1531-2013 http://hdl.handle.net/10803/124220 ADVERTIMENT. L'accés als continguts d'aquesta tesi doctoral i la seva utilització ha de respectar els drets de la persona autora. Pot ser utilitzada per a consulta o estudi personal, així com en activitats o materials d'investigació i docència en els termes establerts a l'art. 32 del Text Refós de la Llei de Propietat Intel·lectual (RDL 1/1996). Per altres utilitzacions es requereix l'autorització prèvia i expressa de la persona autora. En qualsevol cas, en la utilització dels seus continguts caldrà indicar de forma clara el nom i cognoms de la persona autora i el títol de la tesi doctoral. No s'autoritza la seva reproducció o altres formes d'explotació efectuades amb finalitats de lucre ni la seva comunicació pública des d'un lloc aliè al servei TDX. Tampoc s'autoritza la presentació del seu contingut en una finestra o marc aliè a TDX (framing). Aquesta reserva de drets afecta tant als continguts de la tesi com als seus resums i índexs. ADVERTENCIA. El acceso a los contenidos de esta tesis doctoral y su utilización debe respetar los derechos de la persona autora. Puede ser utilizada para consulta o estudio personal, así como en actividades o materiales de investigación y docencia en los términos establecidos en el art. 32 del Texto Refundido de la Ley de Propiedad Intelectual (RDL 1/1996). Para otros usos se requiere la autorización previa y expresa de la persona autora. -
Approaches to Bridged Annulenes Using Both Classical and Reactive Intermediates. the Synthesis of the First Diatropic Bridged Th
APPROACHES TO BRIDGED ANNULENES USING BOTH CLASSICAL AND REACTIVE INTERMEDIATES. THE SYNTHESIS OF THE FIRST DIATROPIC BRIDGED THIAANNULENE AND SEVERAL FUSED DIHYDROPYRENES by VIVEKANANTAN S. HER B.Sc., Madurai Kamaraj University, IN D IA 1984 M.Sc., Indian Institute of Technology, Bombay, INDIA 1986 A Dissertation Submitted in Partial Fulfilment of the Requirements for the Degree of DOCTOR OF PHILOSOPHY ill the Department of Chemistry We accept this dissertation as conforming to the required standard Dr. R. H. M itchell Dr. A. Fischer Dr. T. M. Fyles Dr. E. E. Ishiguro Dr. R. V. W illiams © VIVEKANANTAN S. IYER, 1994 University of Victoria All rights reserved. This dissertation may not he reproduced in whole or in part, by mimeograph or by any other means without the permission of the author. 11 Supervisor: Professor Dr. R. H. Mitchell ABSTRACT The successful synthesis of the first bri~ged thia[13]annulene, trans-9b,9c dimethyl-9b,9c-dihydrophenyleno[l,9-bc)thiophene, 120, was achieved in 11 steps, starting from 3-methylthiophene, 111. Using the external and internal proton chemical shifts of 120, it was shown unambiguously to be the first diatropic bridged thia annulene. From the proton chemical shifts of 120, its diatropicity was estimated to be about 35-40% that of dimethyldihydropyrene 12. Synthesis of the potential intermediate 2,4-bis(bromomethyl)-3-methylthiophene, 110, is expected to lead to synthese;, of a variety of new bridged annulenes. Synthesis of the quasi-biphenyiene, 155, was attempted. The precursor to 155, 1,3-bis(methoxymethyl}-2-methylbiphenylene, 170, was synthesised from 1,2- dibromobenzene, 82, in 4 steps. -
7 Benzene and Aromatics
Benzene and Aromatic Compounds Chapter 15 Organic Chemistry, 8th Edition John McMurry 1 Background • Benzene (C6H6) is the simplest aromatic hydrocarbon (or arene). • Four degrees of unsaturation. • It is planar. • All C—C bond lengths are equal. • Whereas unsaturated hydrocarbons such as alkenes, alkynes and dienes readily undergo addition reactions, benzene does not. • Benzene reacts with bromine only in the presence of FeBr3 (a Lewis acid), and the reaction is a substitution, not an addition. 2 The Structure of Benzene: MO 3 The Structure of Benzene: Resonance The true structure of benzene is a resonance hybrid of the two Lewis structures. 4 Aromaticity – Resonance Energy 5 Stability of Benzene - Aromaticity Benzene does not undergo addition reactions typical of other highly unsaturated compounds, including conjugated dienes. 6 The Criteria for Aromaticity Four structural criteria must be satisfied for a compound to be aromatic. [1] A molecule must be cyclic. 7 The Criteria for Aromaticity [2] A molecule must be completely conjugated (all atoms sp2). 8 The Criteria for Aromaticity [3] A molecule must be planar. 9 The Criteria for Aromaticity—Hückel’s Rule [4] A molecule must satisfy Hückel’s rule. 10 The Criteria for Aromaticity—Hückel’s Rule 1. Aromatic—A cyclic, planar, completely conjugated compound with 4n + 2 π electrons. 3. Antiaromatic—A cyclic, planar, completely conjugated compound with 4n π electrons. 5. Not aromatic (nonaromatic)—A compound that lacks one (or more) of the following requirements for aromaticity: being cyclic, -
Annulenes, Barrelene, Aromatic Ions and Antiaromaticity
Annulenes, Barrelene, Aromatic Ions and Antiaromaticity Annulenes Monocyclic compounds made up of alternating conjugated double bonds are called annulenes. Benzene and 1,3,5,7-cyclooctatetraene are examples of annulenes; they are named [6]annulene and [8]annulene respectively, according to a general nomenclature system in which the number of pi-electrons in an annulene is designated by a number in brackets. Some annulenes are aromatic (e.g. benzene), but many are not due to non- planarity or a failure to satisfy the Hückel Rule. Compounds classified as [10]annulenes (a Hückel Rule system) serve to illustrate these factors. As shown in the following diagram, 1,3,5,7,9-cyclodecapentaene fails to adopt a planar conformation, either in the all cis-configuration or in its 1,5-trans-isomeric form. The transannular hydrogen crowding that destabilizes the latter may be eliminated by replacing the interior hydrogens with a bond or a short bridge (colored magenta in the diagram). As expected, the resulting 10 π-electron annulene derivatives exhibit aromatic stability and reactivity as well as characteristic ring current anisotropy in the nmr. Naphthalene and azulene are [10]annulene analogs stabilized by a transannular bond. Although the CH2bridged structure to the right of naphthalene in the diagram is not exactly planar, the conjugated 10 π-electron ring is sufficiently close to planarity to achieve aromatic stabilization. The bridged [14]annulene compound on the far right, also has aromatic properties. Barrelene Formulation of the Hückel rule prompted organic chemists to consider the possible aromaticity of many unusual unsaturated hydrocarbons. One such compound was the 6 π- electron bicyclic structure, now known as barrelene. -
Chapter 12 Arenes and Aromaticity
CH. 12 Chapter 12 Arenes and Aromaticity Arenes Arenes are hydrocarbon derivatives of benzene. They are called aromatic systems due to their special stability (not due to their aroma!). The special stability results from the highly conjugated system with the electrons delocalized all the way around the ring. The name benzene comes from the Arabic luban jawi or “incense from Java” since it is isolated as a degradation product of gum benzoin. This is a balsam obtained from a tree that grows in Java and Sumatra. This degradation product is benzoic acid, which can be decarboxylated by heating with calcium oxide, CaO. O C OH CaO + CO2 heat benzoic acid benzene And tolu balsam from the South American tolu tree, when distilled, produces toluene or methylbenzene. The term aliphatic hydrocarbons, given to alkanes, alkenes, alkynes and benzene derivatives, comes from the Greek word aleiphar which means oil or unguent. Benzene Benzene has three double bonds that are conjugated and in a circular arrangement. Due to this conjugation and circular arrangement, the double bonds are much less reactive than normal, isolated alkenes. For example, benzene will not react with bromine or hydrogen gas in the presence of a metal catalyst, reactions that alkenes undergo readily. Br H Br2 CH3 CH CH CH3 CH3 C C CH3 rt H Br Br2 No Reaction rt 1 CH. 12 H2, Pd CH3 CH CH CH3 CH3CH2CH2CH3 rt H , Pd 2 No Reaction rt If we examine the structure of benzene we do not see a series of alternating double and single bonds as we would expect from the Lewis structure. -
How Do the Hückel and Baird Rules Fade Away in Annulenes?
molecules Article How do the Hückel and Baird Rules Fade away in Annulenes? Irene Casademont-Reig 1,2 , Eloy Ramos-Cordoba 1,2 , Miquel Torrent-Sucarrat 1,2,3 and Eduard Matito 1,3* 1 Donostia International Physics Center (DIPC), 20018 Donostia, Euskadi, Spain; [email protected] (I.C.-R.); [email protected] (E.R.-C.); [email protected] (M.T.-S.) 2 Kimika Fakultatea, Euskal Herriko Unibertsitatea (UPV/EHU), 20080 Donostia, Euskadi, Spain 3 IKERBASQUE, Basque Foundation for Science, 48013 Bilbao, Euskadi, Spain * Correspondence: [email protected]; Tel.: +34-943018513 Academic Editors: Diego Andrada and Israel Fernández Received: 20 December 2019; Accepted: 29 January 2020; Published: 7 February 2020 Abstract: Two of the most popular rules to characterize the aromaticity of molecules are those due to Hückel and Baird, which govern the aromaticity of singlet and triplet states. In this work, we study how these rules fade away as the ring structure increases and an optimal overlap between p orbitals is no longer possible due to geometrical restrictions. To this end, we study the lowest-lying singlet and triplet states of neutral annulenes with an even number of carbon atoms between four and eighteen. First of all, we analyze these rules from the Hückel molecular orbital method and, afterwards, we perform a geometry optimization of the annulenes with several density functional approximations in order to analyze the effect that the distortions from planarity produce on the aromaticity of annulenes. Finally, we analyze the performance of three density functional approximations that employ different percentages of Hartree-Fock exchange (B3LYP, CAM-B3LYP and M06-2X) and Hartree-Fock. -
Hydrocarbon Chains and Rings: Bond Length Alternation in Finite Molecules
Theor Chem Acc (2015) 134:114 DOI 10.1007/s00214-015-1709-4 REGULAR ARTICLE Hydrocarbon chains and rings: bond length alternation in finite molecules Jeno˝ Kürti1 · János Koltai1 · Bálint Gyimesi1 · Viktor Zólyomi2,3 Received: 19 June 2015 / Accepted: 28 July 2015 © Springer-Verlag Berlin Heidelberg 2015 Abstract We present a theoretical study of Peierls distor- more recent publications about the BLA in carbon chains, tion in carbon rings. We demonstrate using the Longuet- see, e.g. Refs. [3–6] and references therein. Polyyne in par- Higgins–Salem model that the appearance of bond alterna- ticular remains a hot topic experimentally and theoretically, tion in conjugated carbon polymers is independent of the in part due to the difficulty in determining the band gap of boundary conditions and does in fact appear in carbon rings this material [6]. One reason why it is difficult to correctly just as in carbon chains. We use the Hartree–Fock approxi- calculate the band gap in Peierls distorted systems is that mation and density functional theory to show that this the bond length alternation directly determines the band behaviour is retained at the first principles level. gap, i.e. if the bond length alternation is underestimated (as is often the case in, e.g. density functional theory), the band Keywords Peierls distortion · Conjugated polymers · gap will be underestimated as well. As such, the matter of Annulenes · Longuet-Higgins–Salem model · Density correctly describing bond length alternation is a current and functional theory important topic. In addition, Peierls distortion brings with it some inter- esting fundamental questions, such as whether the bond 1 Introduction length alternation appears in carbon rings. -
Synthetic and Theoretical Studies of Cyclobuta[1,2:3,4]Dicyclopentene
Synthetic and Theoretical Studies of Cyclobuta[1,2:3,4]dicyclopentene. Organocobalt Intermediates in the Construction of the Unsaturated Carbon Skeleton and Their Transformation into Novel Cobaltacyclic Complexes by C-C Insertion Andrew G. Myers,* Miki Sogi, Michael A. Lewis, and Stephen P. Arvedson Department of Chemistry and Chemical Biology, Harvard University, Cambridge, Massachusetts 02138 [email protected] Received December 3, 2003 Theoretical and synthetic studies of the tricyclic 10π-electron hydrocarbon cyclobuta[1,2:3,4]- dicyclopentene (1), a nominally aromatic structure that has never been synthesized, are described. Geometry optimization by density-functional-theory calculations (B3LYP/6-31G(d,p)) predict that 1 is a D2h symmetric structure with nonalternant C-C single and double bonds. The calculations also predict that 1 is 4.7 kcal/mol higher in energy than the isomeric hydrocarbon 1,6-didehydro- [10]annulene (2), a molecule known to isomerize to 1,5-didehydronaphthalene (4) above -50 °C. Calculated enthalpic changes of homodesmotic reactions support the notion that 1 is an aromatic molecule with a resonance stabilization energy (RSE) about half to two-thirds that of benzene on a per-molecule basis. Investigations of potential synthetic pathways to 1 initially utilized as starting material the tricyclic carbonate 11, the product of an intramolecular [2 + 2]-photocyclization reaction. In these studies, 11 was transformed in several steps to the distannane 12, which upon treatment with boron fluoride ethyl etherate is believed to have formed the unstable hydrocarbon bicyclopentadienylidene (13). In an effort to avoid cleavage of the central, four-membered ring of unsaturated tricyclo[5.3.0.02,6]decane intermediates (perhaps the result of 10-electron electrocyclic ring opening of the tetraene 8), synthetic approaches to 1 employing cobalt-cyclobutadiene complexes 18 and 19 were pursued. -
Aromaticity and Aromatic Compounds
Aromaticity Benzene- highly unsaturated compound (4 degrees of unsaturation). Resonance structures are also called Kekule' structures: or or Benzene is an aromatic compound- low H/C ratio and has a fragrance Benzene does not behave like an unsaturated compound: does not add Br2 or [ox] with KMnO4 does not add water does not add H2 except under forcing conditions Br2 CH2Cl2 H+ no reaction H2O H2 Pd MeOH Also KMnO4 does not react with benzene. Benzene does react with Br2 in the presence of a catalyst but gives substitution, not addition Br no Br2 Br2 reaction CH2Cl2 FeBr3 Tendency to undergo substitution instead of addition is another characteristic of aromatic compounds. Cyclooctatetraene adds Br2 readily and it reacts with KMnO4 cyclooctatetraene is not aromatic Br Br2 Br 1 equiv. + conjugate isomers cyclooctatetraene Alternating single and double bonds in a ring is not necessarily aromatic. Page 1 Aromaticity Stability of Benzene- from heats of hydrogenation 1,3,5-cyclohexatriene + H2 E resonance energy due to conjugation ΔH° benzene 8 kJ -360 kJ/mol predicted cyclohexene ΔH° -208 kJ/mol ΔH° -120 kJ/mol -240 kJ/mol -232 kJ/mol Resonance energy = (-360 kJ) - (-208 kJ) = 152 kJ Resonance energy- difference between energy released for hydrogenation of benzene and the predicted value for hydrogenation of 1,3,5 cyclohexatriene Resonance approach and M.O. approach ¾ Resonance theory shows that benzene has two Lewis structures double-headed arrow is used (not equilibrium arrows!) not an equilibrium! More than one equivalent resonance structure implies resonance stabilization. C-C bond length is 1.39 angstroms Approximately halfway between C-C single bond (1.47) and C=C bond (1.33) Page 2 Aromaticity ¾ M.O Approach … we combine p orbitals … Each carbon is sp2 hybridized so the C-C-C bond angles are all 120° Therefore, benzene is planar. -
Aromatic Compounds
Aromatic Compounds Historically, benzene and its first derivatives had pleasant aromas, and were called aromatic compounds. Structure of Benzene Kekulé Structure Kekulé (1866) bravely proposed that benzene had a cyclic structure with three alternating C=C double and three C-C single bonds. H H C H C C C C H C H H Whilst this is reasonably close to accurate, it cannot be exactly correct since this would require that 1,2- dichlorobenzene existed as two isomeric forms, yet it was known that it did not. Ch16 Aromatic Compounds (landscape).docx Page 1 Resonance Structure The Kekulé structure would have the single bonds of longer length than the double bonds, and thus an irregular hexagonal shape. But spectroscopy had shown that benzene had a planar ring, with all the carbon-carbon bond distances the same 1.397Å (C-C typically 1.48Å, C=C typically 1.34Å). Since the atoms are the same distance apart, and the only difference is the location of the electrons in the two Kekulé structures, they are in fact resonance structures of one another. This implies that the bond order should be 1.5, and that the electrons are delocalized around the ring. Because of the delocalization of the electrons, often the double bonds are represented by a circle in the middle of the hexagon. Ch16 Aromatic Compounds (landscape).docx Page 2 This resonance description lets us draw a more realistic representation of benzene, with 6 sp2 hybrid carbons, each bonded to one hydrogen atom. All the carbon-carbon bonds are of equal length, and all the bond angles are 120°. -
12: Conjugated and Aromatic Molecules
(3/94)(9,10/96)(3,4,5/04) Neuman Chapter 12 Chapter 12 Conjugated and Aromatic Molecules from Organic Chemistry by Robert C. Neuman, Jr. Professor of Chemistry, emeritus University of California, Riverside [email protected] <http://web.chem.ucsb.edu/~neuman/orgchembyneuman/> Chapter Outline of the Book ************************************************************************************** I. Foundations 1. Organic Molecules and Chemical Bonding 2. Alkanes and Cycloalkanes 3. Haloalkanes, Alcohols, Ethers, and Amines 4. Stereochemistry 5. Organic Spectrometry II. Reactions, Mechanisms, Multiple Bonds 6. Organic Reactions *(Not yet Posted) 7. Reactions of Haloalkanes, Alcohols, and Amines. Nucleophilic Substitution 8. Alkenes and Alkynes 9. Formation of Alkenes and Alkynes. Elimination Reactions 10. Alkenes and Alkynes. Addition Reactions 11. Free Radical Addition and Substitution Reactions III. Conjugation, Electronic Effects, Carbonyl Groups 12. Conjugated and Aromatic Molecules 13. Carbonyl Compounds. Ketones, Aldehydes, and Carboxylic Acids 14. Substituent Effects 15. Carbonyl Compounds. Esters, Amides, and Related Molecules IV. Carbonyl and Pericyclic Reactions and Mechanisms 16. Carbonyl Compounds. Addition and Substitution Reactions 17. Oxidation and Reduction Reactions 18. Reactions of Enolate Ions and Enols 19. Cyclization and Pericyclic Reactions *(Not yet Posted) V. Bioorganic Compounds 20. Carbohydrates 21. Lipids 22. Peptides, Proteins, and α−Amino Acids 23. Nucleic Acids ************************************************************************************** *Note: Chapters marked with an (*) are not yet posted. 0 (3/94)(9,10/96)(3,4,5/04) Neuman Chapter 12 12: Conjugated and Aromatic Molecules 12.1 Conjugated Molecules 12-4 1,3-Butadiene (12.1A) 12-4 Atomic Orbital Overlap in 1,3-Butadiene Molecular Orbitals The Bonding M.O.'s Conformations Other Alternating Multiple Bonds Pentadienes (12.1B) 12-7 1,3-Pentadiene 1,4-Pentadiene 1,2-Pentadiene. -
Hückel and Möbius Annulenes: Relationships Between Their Hückel-Level Orbitals and Their Hückel-Level Energies
EDUCAÇÃO HÜCKEL AND MÖBIUS ANNULENES: RELATIONSHIPS BETWEEN THEIR HÜCKEL-LEVEL ORBITALS AND THEIR HÜCKEL-LEVEL ENERGIES Richard Francis Langler Department of Chemistry, Mount Allison University, Sackville, New Brunswick, Canada E4L 1G8 Guillermo Salgado and Carlos Mendizabal Universidad Santo Tomas, Agropecuaria, Av. Ejercito 146, Santiago, Chile Recebido em 28/10/99; aceito em 29/2/00 The problem of convenient access to quantitative Hückel-level descriptions of Möbius and Hückel annulenes for undergraduate lectures about aromaticity is discussed. Frost circle, Zimmerman circle, double circle and Langler semicircular mnemonics are described. The relationship between spectra (complete sets of secular equation roots) for an isoconjugate pair of Hückel and Möbius annulenes and the corresponding acyclic polyene with one less carbon is fully developed. In addi- tion to providing an alternative path to exact spectrum roots, this relationship provides immediate access to almost half of the eigenfunctions for an isoconjugate annulene pair. The remaining eigenfunctions may be obtained very easily. Keywords: aromaticity; circle mnemonics; Hückel theory. INTRODUCTION In 1931, Hückel described a very simple molecular orbital approach to the π-electronic structure of planar molecules1. Since then, many textbooks have been written which introduce Hückel theory to undergraduate students and apply it, prima- rily, to planar hydrocarbons (see reference 2 for some repre- sentative texts). Furthermore, many concerted chemical reac- tions (e.g. Diels-Alder)