Constraining Differential Rotation of Sun-Like Stars from Asteroseismic and Starspot Rotation Periods
Total Page:16
File Type:pdf, Size:1020Kb
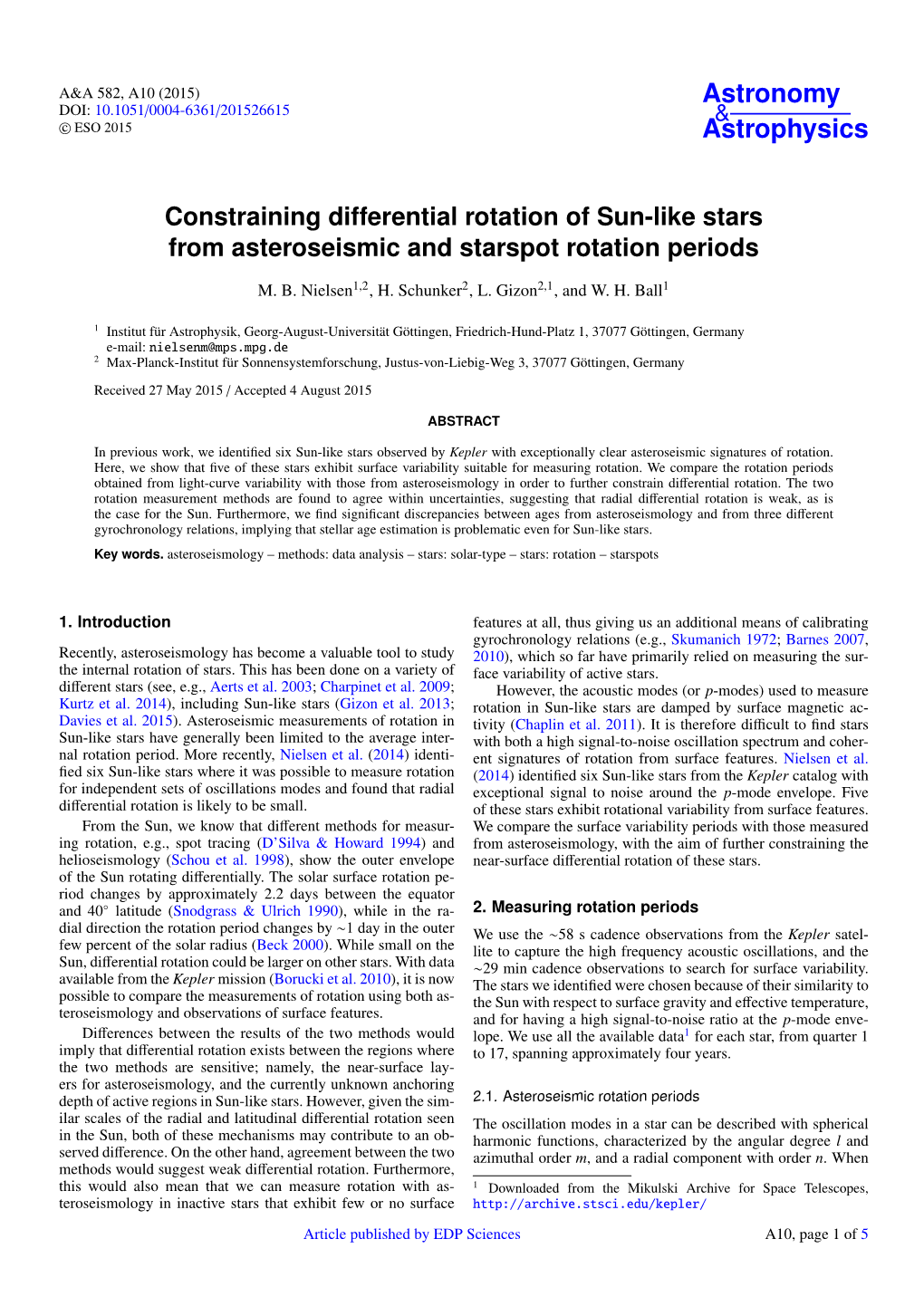
Load more
Recommended publications
-
Young Nearby Stars by Adam Conrad Schneider (Under The
Young Nearby Stars by Adam Conrad Schneider (Under the Direction of Professor Inseok Song) Abstract Nearby young stars are without equal as stellar and planetary evolution laboratories. The aim of this work is to use age diagnostic considerations to execute a complete survey for new nearby young stars and to efficiently reevaluate and constrain their ages. Because of their proximity and age, young, nearby stars are the most desired targets for any astrophysical study focusing on the early stages of star and planet formation. Identifying nearby, young, low-mass stars is challenging because of their inherent faint- ness and age diagnostic degeneracies. A new method for identifying these objects has been developed, and a pilot study of its effectiveness is demonstrated by the identification of two definite new members of the TW Hydrae Association. Nearby, young, solar-type stars are initially identified in this work by their fractional X-ray luminosity. The results of a large-scale search for nearby, young, solar-type stars is presented. Follow-up spectroscopic observations are taken in order to measure various age diagnostics in order to accurately assess stellar ages. Age, one of the most fundamental properties of a star, is also one of the most difficult to determine. While a variety of proce- dures have been developed and utilized to approximate ages for solar-type stars, with varying degrees of success, a comprehensive age-dating technique has yet to be constructed. Often- times, different methods exhibit contradictory or conflicting findings. Such inconsistencies demonstrate the value of a uniform method of determining stellar ages. -
Age Dating the Galactic Bar with the Nuclear Stellar Disc
MNRAS 492, 4500–4511 (2020) doi:10.1093/mnras/staa140 Advance Access publication 2020 January 20 Age dating the Galactic bar with the nuclear stellar disc Downloaded from https://academic.oup.com/mnras/article-abstract/492/3/4500/5709929 by Institute of Child Health/University College London user on 14 February 2020 Junichi Baba1‹ and Daisuke Kawata 2 1National Astronomical Observatory of Japan, Mitaka, Tokyo 181-8588, Japan 2Mullard Space Science Laboratory, University College London, Holmbury St. Mary, Dorking, Surrey, RH5 6NT, UK Accepted 2020 January 11. Received 2019 December 26; in original form 2019 September 16 ABSTRACT From the decades of the theoretical studies, it is well known that the formation of the bar triggers the gas funnelling into the central sub-kpc region and leads to the formation of a kinematically cold nuclear stellar disc (NSD). We demonstrate that this mechanism can be used to identify the formation epoch of the Galactic bar, using an N-body/hydrodynamics simulation of an isolated Milky Way–like galaxy. As shown in many previous literature, our simulation shows that the bar formation triggers an intense star formation for ∼1 Gyr in the central region and forms an NSD. As a result, the oldest age limit of the NSD is relatively sharp, and the oldest population becomes similar to the age of the bar. Therefore, the age distribution of the NSD tells us the formation epoch of the bar. We discuss that a major challenge in measuring the age distribution of the NSD in the Milky Way is contamination from other non-negligible stellar components in the central region, such as a classical bulge component. -
Ages of Young Stars
Ages of Young Stars David R. Soderblom Space Telescope Science Institute, Baltimore MD USA Lynne A. Hillenbrand Caltech, Pasadena CA USA Rob. D. Jeffries Astrophysics Group, Keele University, Staffordshire, ST5 5BG, UK Eric E. Mamajek Department of Physics & Astronomy, University of Rochester, Rochester NY, 14627-0171, USA Tim Naylor School of Physics, University of Exeter, Stocker Road, Exeter EX4 4QL, UK Determining the sequence of events in the formation of stars and planetary systems and their time-scales is essential for understanding those processes, yet establishing ages is fundamentally difficult because we lack direct indicators. In this review we discuss the age challenge for young stars, specifically those less than ∼100 Myr old. Most age determination methods that we discuss are primarily applicable to groups of stars but can be used to estimate the age of individual objects. A reliable age scale is established above 20 Myr from measurement of the Lithium Depletion Boundary (LDB) in young clusters, and consistency is shown between these ages and those from the upper main sequence and the main sequence turn-off – if modest core convection and rotation is included in the models of higher-mass stars. Other available methods for age estimation include the kinematics of young groups, placing stars in Hertzsprung-Russell diagrams, pulsations and seismology, surface gravity measurement, rotation and activity, and lithium abundance. We review each of these methods and present known strengths and weaknesses. Below ∼ 20 Myr, both model-dependent and observational uncertainties grow, the situation is confused by the possibility of age spreads, and no reliable absolute ages yet exist. -
Characterization of Dusty Debris Disks: the IRAS and Hipparcos Catalogs
Characterization of Dusty Debris Disks: The IRAS and Hipparcos Catalogs Joseph H. Rhee1, Inseok Song1 B. Zuckerman2, and Michael McElwain2 Received ; accepted To appear in ApJ, 2007 arXiv:astro-ph/0609555v4 6 Feb 2007 1Gemini Observatory, 670 North A’ohoku Place, Hilo, HI 96720; [email protected], [email protected] 2Department of Physics and Astronomy and NASA Astrobiology Institute UCLA, Los Angeles, CA 90095; [email protected], [email protected] –2– ABSTRACT Dusty debris disks around main-sequence stars are signposts for the existence of planetesimals and exoplanets. From cross-correlating Hipparcos stars with the IRAS catalogs, we identify 146 stars within 120 pc of Earth that show excess emission at 60 µm. This search took special precautions to avoid false positives. Our sample is reasonably well distributed from late B to early K-type stars, but it contains very few later type stars. Even though IRAS flew more than 20 years ago and many astronomers have cross-correlated its catalogs with stellar catalogs, we were still able to newly identify debris disks at as many as 33 main-sequence stars; of these, 32 are within 100 pc of Earth. The power of an all-sky survey satellite like IRAS is evident when comparing our 33 new debris disks with the total of only 22 dusty debris disk stars detected first with the more sensitive, but pointed, satellite ISO . Our investigation focuses on the mass, dimensions, and evolution of dusty debris disks. Subject headings: infrared: stars — circumstellar matter — planetary systems: formation — Kuiper Belt –3– 1. Introduction Dusty debris disks that surround nearby main-sequence stars were first detected by the Infrared Astronomical Satellite (IRAS ) in 1983. -
Observed Sizes of Planet-Forming Disks Trace Viscous Spreading
UvA-DARE (Digital Academic Repository) Observed sizes of planet-forming disks trace viscous spreading Trapman, L.; Rosotti, G.; Bosman, A.D.; Hogerheijde, M.R.; van Dishoeck, E.F. DOI 10.1051/0004-6361/202037673 Publication date 2020 Document Version Final published version Published in Astronomy & Astrophysics Link to publication Citation for published version (APA): Trapman, L., Rosotti, G., Bosman, A. D., Hogerheijde, M. R., & van Dishoeck, E. F. (2020). Observed sizes of planet-forming disks trace viscous spreading. Astronomy & Astrophysics, 640, [A5]. https://doi.org/10.1051/0004-6361/202037673 General rights It is not permitted to download or to forward/distribute the text or part of it without the consent of the author(s) and/or copyright holder(s), other than for strictly personal, individual use, unless the work is under an open content license (like Creative Commons). Disclaimer/Complaints regulations If you believe that digital publication of certain material infringes any of your rights or (privacy) interests, please let the Library know, stating your reasons. In case of a legitimate complaint, the Library will make the material inaccessible and/or remove it from the website. Please Ask the Library: https://uba.uva.nl/en/contact, or a letter to: Library of the University of Amsterdam, Secretariat, Singel 425, 1012 WP Amsterdam, The Netherlands. You will be contacted as soon as possible. UvA-DARE is a service provided by the library of the University of Amsterdam (https://dare.uva.nl) Download date:24 Sep 2021 A&A 640, A5 (2020) Astronomy https://doi.org/10.1051/0004-6361/202037673 & © ESO 2020 Astrophysics Observed sizes of planet-forming disks trace viscous spreading L. -
Ages of Young Stars1
arXiv:1311.7024v1 [astro-ph.SR] 27 Nov 2013 0 Accepted for publication as a chapter in Protostars and Planets VI University of Arizona Press (2014) eds. H. Beuther, R. Klessen, C. Dullemond, Th. Henning. 1 Ages of Young Stars1 David R. Soderblom Space Telescope Science Institute, Baltimore MD USA Lynne A. Hillenbrand Caltech, Pasadena CA USA Rob. D. Jeffries Astrophysics Group, Keele University, Staffordshire, ST5 5BG, UK Eric E. Mamajek Department of Physics & Astronomy, University of Rochester, Rochester NY, 14627-0171, USA Tim Naylor School of Physics, University of Exeter, Stocker Road, Exeter EX4 4QL, UK Determining the sequence of events in the formation of stars and planetary systems and their time-scales is essential for understanding those processes, yet establishing ages is fundamentally difficult because we lack direct indicators. In this review we discuss the age challenge for young stars, specifically those less than ∼100 Myr old. Most age determination methods that we discuss are primarily applicable to groups of stars but can be used to estimate the age of individual objects. A reliable age scale is established above 20 Myr from measurement of the Lithium Depletion Boundary (LDB) in young clusters, and consistency is shown between these ages and those from the upper main sequence and the main sequence turn-off – if modest core convection and rotation is included in the models of higher-mass stars. Other available methods for age estimation include the kinematics of young groups, placing stars in Hertzsprung-Russell diagrams, pulsations and seismology, surface gravity measurement, rotation and activity, and lithium abundance. We review each of these methods and present known strengths and weaknesses. -
Age Dating the Galactic Bar with the Nuclear Stellar Disc
MNRAS 000, 000–000 (0000) Preprint 15 January 2020 Compiled using MNRAS LATEX style file v3.0 Age Dating the Galactic Bar with the Nuclear Stellar Disc Junichi Baba1⋆, and Daisuke Kawata2 1 National Astronomical Observatory of Japan, Mitaka, Tokyo 181-8588, Japan 2 Mullard Space Science Laboratory, University College London, Holmbury St. Mary, Dorking, Surrey, RH5 6NT, UK Accepted 2020 January 11. Received 2019 December 26; in original form 2019 September 17 ABSTRACT From the decades of the theoretical studies, it is well known that the formation of the bar triggers the gas funnelling into the central sub-kpc region and leads to the formation of a kinematically cold nuclear stellar disc (NSD). We demonstrate that this mechanism can be used to identify the formation epoch of the Galactic bar, using an N-body/hydrodynamics simulation of an isolated Milky Way-like galaxy. As shown in many previous literature, our simulation shows that the bar formation triggers an intense star formation for ∼ 1 Gyr in the central region, and forms a NSD. As a result, the oldest age limit of the NSD is relatively sharp, and the oldest population becomes similar to the age of the bar. Therefore, the age distribution of the NSD tells us the formation epoch of the bar. We discuss that a major challenge in measuring the age distribution of the NSD in the Milky Way is contamination from other non-negligible stellar components in the central region, such as a classical bulge component. We demonstrate that because the NSD is kinematically colder than the other stellar populations in the Galactic central region, the NSD population can be kinematically distinguished from the other stellar populations, if the 3D velocity of tracer stars are accurately measured. -
AI for Dating Stars: a Benchmarking Study for Gyrochronology
AI for dating stars: a benchmarking study for gyrochronology Andres´ Moya Jarmi Recio-Mart´ınez Roberto J. Lopez-Sastre´ Universidad Politecnica´ de Madrid University of Alcala´ University of Alcala´ [email protected] [email protected] [email protected] Abstract using diverse methods. Soderblom presented two excellent surveys describing most of these techniques [54, 55]. In astronomy, age is one of the most difficult stellar prop- Within the group of approaches for stellar dating, one of erties to measure, and gyrochronology is one of the most the most promising statistical techniques is gyrochronology promising techniques for the task. It consists in dating or dating stars using their rotational period. Stars are born stars using their rotational period and empirical linear re- with a range of rotational velocities for different physical lations with other observed stellar properties, such as stel- reasons, see [6]. Then, the process called magnetic braking lar effective temperature, parallax, and/or photometric col- reduces the rotational velocity of the star with time [49]. ors in different passbands, for instance. However, these ap- Since late 60’s [10, 26, 37, 38, 59], we know that rotational proaches do not allow to reproduce all the observed data, braking, or braking law, is a function of the rotational pe- resulting in potential significant deviations in age estima- riod. That is, the larger the rotation, the larger the braking. tion. In this context, we propose to explore the stellar dat- Therefore, with time, all the stars tend to converge to a sim- ing problem using gyrochronology from the AI perspective. -
Statistical Analyses of Massive Stars and Stellar Populations
Statistical Analyses of Massive Stars and Stellar Populations Dissertation zur Erlangung des Doktorgrades (Dr. rer. nat.) der Mathematisch-Naturwissenschaftlichen Fakultät der Rheinischen Friedrich-Wilhelms-Universität Bonn vorgelegt von Fabian Schneider aus Duisburg Bonn, Oktober 2014 Angefertigt mit Genehmigung der Mathematisch-Naturwissenschaftlichen Fakultät der Rheinischen Friedrich-Wilhelms-Universität Bonn 1. Gutachter: Prof. Dr. Norbert Langer 2. Gutachter: Prof. Dr. Robert G. Izzard Tag der Promotion: 11. Februar 2015 Erscheinungsjahr: 2015 I, a universe of atoms, an atom in the universe. (Richard P. Feynman) Abstract Massive stars, i.e. stars more massive than about ten times that of the Sun, are key agents in the Universe. They synthesise many of the chemical elements that are so important for life on Earth, helped reionising the early Universe and end their lives in spectacular supernova explosions that are visible out to large distances. Because of their important role for much of astrophysics, accurate and reliable stellar evolution models are essential. However, recent developments regarding wind mass loss rates, internal mixing processes and duplicity seriously challenge our understanding of massive stars and stellar populations. It is now established that most, if not all, massive stars reside in binaries or higher order multiple systems such that more than two-thirds of all massive stars are expected to interact through mass transfer with a binary companion during their lives. We investigate the con- sequences of this finding for coeval stellar populations and show that the most massive stars in star clusters are likely all rejuvenated binary products that may seriously bias the determination of cluster ages. We further find that wind mass loss from stars and binary mass transfer leave their fingerprints in the high mass end of stellar mass functions. -
The Ages of Stars
AA48CH15-Soderblom ARI 16 July 2010 22:29 The Ages of Stars David R. Soderblom Space Telescope Science Institute, Baltimore, MD 21218; email: [email protected] Annu. Rev. Astron. Astrophys. 2010. 48:581–629 Key Words First published online as a Review in Advance on galaxy: open clusters and associations; stars: ages, evolution, low-mass, May 25, 2010 pre-main sequence, solar-type The Annual Review of Astronomy and Astrophysics is online at astro.annualreviews.org Abstract This article’s doi: The age of an individual star cannot be measured, only estimated through 10.1146/annurev-astro-081309-130806 mostly model-dependent or empirical methods, and no single method works Copyright c 2010 by Annual Reviews. well for a broad range of stellar types or for a full range in age. This review All rights reserved presents a summary of the available techniques for age-dating stars and en- 0066-4146/10/0922-0581$20.00 sembles of stars, their realms of applicability, and their strengths and weak- Annu. Rev. Astron. Astrophys. 2010.48:581-629. Downloaded from www.annualreviews.org nesses. My emphasis is on low-mass stars because they are present from all Access provided by State University of New York - Stony Brook on 08/28/17. For personal use only. epochs of star formation in the Galaxy and because they present both special opportunities and problems. The ages of open clusters are important for understanding the limitations of stellar models and for calibrating empirical age indicators. For individual stars, a hierarchy of quality for the available age-dating methods is described. -
The Evolution of and Starburst-AGN Connection in Luminous and Ultraluminous Infrared Galaxies and Their Link to Globular Cluster Formation
City University of New York (CUNY) CUNY Academic Works All Dissertations, Theses, and Capstone Projects Dissertations, Theses, and Capstone Projects 6-2014 The Evolution of and Starburst-AGN Connection in Luminous and Ultraluminous Infrared Galaxies and their Link to Globular Cluster Formation Stephanie L. Fiorenza Graduate Center, City University of New York How does access to this work benefit ou?y Let us know! More information about this work at: https://academicworks.cuny.edu/gc_etds/206 Discover additional works at: https://academicworks.cuny.edu This work is made publicly available by the City University of New York (CUNY). Contact: [email protected] THE EVOLUTION OF AND STARBURST- AGN CONNECTION IN LUMINOUS AND ULTRALUMINOUS INFRARED GALAXIES AND THEIR LINK TO GLOBULAR CLUSTER FORMATION by Stephanie Lynn Fiorenza A dissertation submitted to the Graduate Faculty in Physics in partial fulfillment of the requirements for the degree of Doctor of Philosophy, The City University of New York 2014 i © 2014 Stephanie Lynn Fiorenza All Rights Reserved ii This manuscript has been read and accepted for the Graduate Faculty in Physics in satisfaction of the dissertation requirement for the degree of Doctor of Philosophy. Dr. Charles T. Liu __________ _____________________ Date Chair of Examining Committee Dr. Steven G. Greenbaum ______________ ____________________________ Date Executive Officer Dr. Alfred Levine Dr. Matthew O’Dowd Dr. Emily Rice Dr. Sivarani Thirupathi ________________________________________________ Supervisory Committee THE CITY UNIVERSITY OF NEW YORK iii Abstract THE EVOLUTION OF AND STARBURST- AGN CONNECTION IN LUMINOUS AND ULTRALUMINOUS INFRARED GALAXIES AND THEIR LINK TO GLOBULAR CLUSTER FORMATION BY STEPHANIE LYNN FIORENZA Advisor: Dr. -