Raman Laser from an Optical Resonator with a Grafted Single Molecule
Total Page:16
File Type:pdf, Size:1020Kb
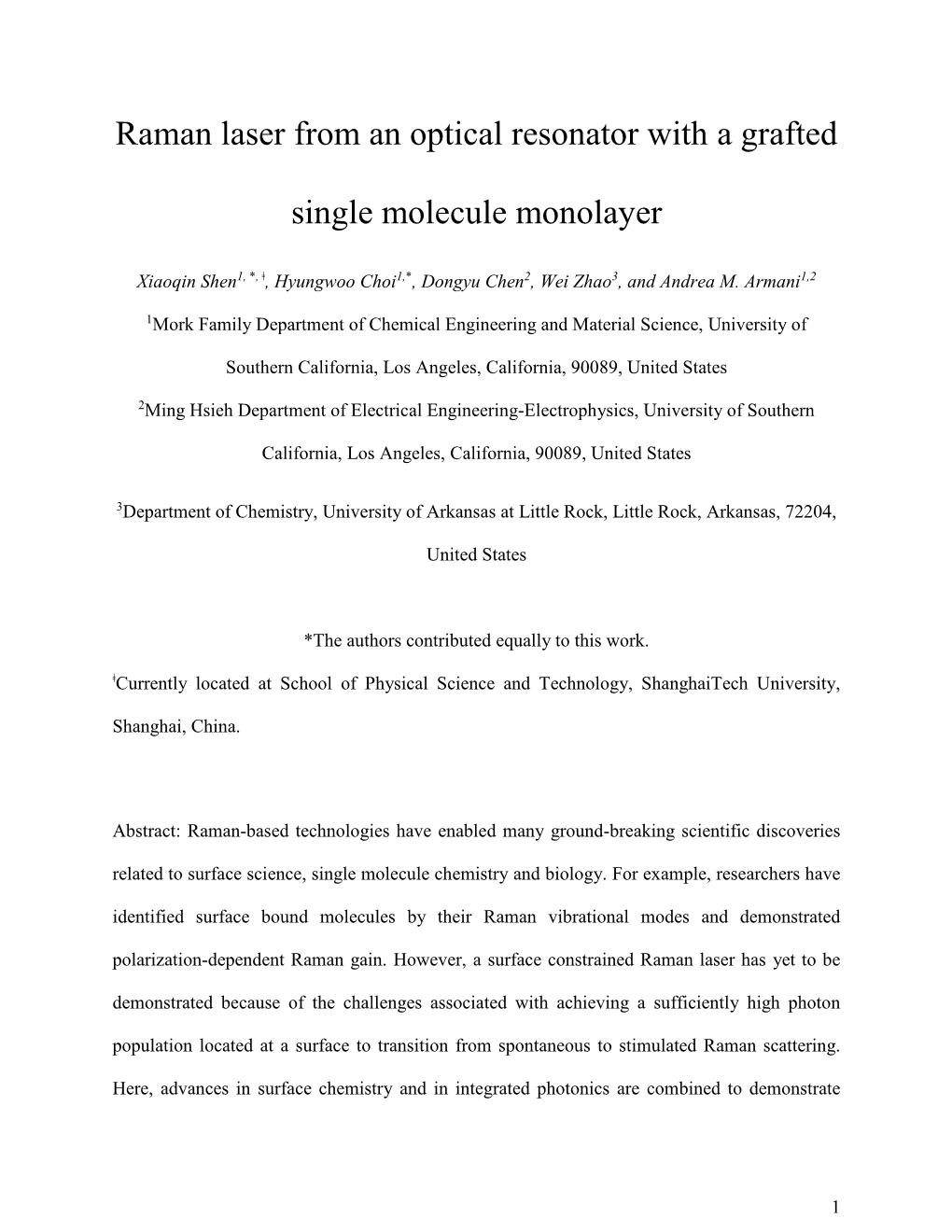
Load more
Recommended publications
-
Passively Q-Switched KTA Cascaded Raman Laser with 234 and 671 Cm−1 Shifts
applied sciences Article Passively Q-Switched KTA Cascaded Raman Laser with 234 and 671 cm−1 Shifts Zhi Xie 1,2, Senhao Lou 2, Yanmin Duan 2, Zhihong Li 2, Limin Chen 1, Hongyan Wang 3, Yaoju Zhang 2 and Haiyong Zhu 2,* 1 College of Mechanical and Electronic Engineering, Fujian Agriculture and Forestry University, Fuzhou 350002, China; [email protected] (Z.X.); [email protected] (L.C.) 2 Wenzhou Key Laboratory of Micro-Nano Optoelectronic Devices, Wenzhou University, Wenzhou 325035, China; [email protected] (S.L.); [email protected] (Y.D.); [email protected] (Z.L.); [email protected] (Y.Z.) 3 Crystech Inc., Qingdao 266100, China; [email protected] * Correspondence: [email protected] Abstract: A compact KTA cascaded Raman system driven by a passively Q-switched Nd:YAG/Cr4+:YAG laser at 1064 nm was demonstrated for the first time. The output spectra with different cavity lengths were measured. Two strong lines with similar intensity were achieved with a 9 cm length cavity. One is the first-Stokes at 1146.8 nm with a Raman shift of 671 cm−1, and the other is the Stokes at 1178.2 nm with mixed Raman shifts of 234 cm−1 and 671 cm−1. At the shorter cavity length of 5 cm, the output Stokes lines with high intensity were still at 1146.8 nm and 1178.2 nm, but the intensity of 1178.2 nm was higher than that of 1146.8 nm. The maximum average output power of 540 mW was obtained at the incident pump power of 10.5 W with the pulse repetition frequency of 14.5 kHz and the pulse width around 1.1 ns. -
Understanding the Application of Raman Spectroscopy to the Detection of Traces of Life
ASTROBIOLOGY Volume 10, Number 2, 2010 ª Mary Ann Liebert, Inc. DOI: 10.1089=ast.2009.0344 Understanding the Application of Raman Spectroscopy to the Detection of Traces of Life Craig P. Marshall,1 Howell G.M. Edwards,2 and Jan Jehlicka3 Abstract Investigating carbonaceous microstructures and material in Earth’s oldest sedimentary rocks is an essential part of tracing the origins of life on our planet; furthermore, it is important for developing techniques to search for traces of life on other planets, for example, Mars. NASA and ESA are considering the adoption of miniaturized Raman spectrometers for inclusion in suites of analytical instrumentation to be placed on robotic landers on Mars in the near future to search for fossil or extant biomolecules. Recently, Raman spectroscopy has been used to infer a biological origin of putative carbonaceous microfossils in Early Archean rocks. However, it has been demonstrated that the spectral signature obtained from kerogen (of known biological origin) is similar to spectra obtained from many poorly ordered carbonaceous materials that arise through abiotic processes. Yet there is still confusion in the literature as to whether the Raman spectroscopy of carbonaceous materials can indeed delineate a signature of ancient life. Despite the similar nature in spectra, rigorous structural interrogation between the thermal alteration products of biological and nonbiological organic materials has not been undertaken. There- fore, we propose a new way forward by investigating the second derivative, deconvolution, and chemometrics of the carbon first-order spectra to build a database of structural parameters that may yield distinguishable characteristics between biogenic and abiogenic carbonaceous material. -
New Exploratory Experiments for Raman Laser Spectroscopy
5 Lasers for Science Facility Programme I Chemistry New exploratory experiments for Raman laser spectroscopy M. Hippler and C. Mohr Department of Chemistry, University of Sheffield, Sheffield, S3 7HF, UK Main contact email address [email protected] Introduction using a modification of the optophone detection [3,4] The analysis of high-resolution rovibrational spectra is introduced by Zare and coworkers. We will also introduce a relevant because it allows the study of the structure and first application, the study of the anharmonic and Coriolis dynamics of molecules and molecular association, and how resonance system of cyclo-propane (C3H6) in the CH- this changes under vibrational excitation. Topics in this stretching region near 3000 cm-1 [5] and present first tentative context are the determination of equilibrium geometries of assignments. [6] isolated molecules and of complexes, the study of the coupling of vibrations, intramolecular vibrational energy Discussion redistribution (IVR), tunnelling and rearrangement To set-up a stimulated laser Raman experiment, a strong processes (for a recent overview, see ref. [1]. Raman Raman pump source at fixed wavelength and a tuneable spectroscopy is an important technique in these studies, stimulating Raman source are required. For this purpose, because it can provide complimentary information which is we modified a Nd:YAG pumped dye laser system not available by IR spectroscopy due to different selection (Continuum Sirah PrecisionScan, NSL4 on loan from the rules and experimental difficulties -
Cobolt Lasers for Raman Spectroscopy – White Paper
Cobolt lasers for Raman Spectroscopy – White Paper 1. Please tell us a bit about Cobolt? Cobolt AB is a Sweden-based manufacturer of high performance diode-pumped lasers and laser diodes in the UV, visible and near-infrared spectral ranges. The company’s laser products serve as key-components in advanced analytical instrumentation equipment for life science, quality control and industrial metrology applications. Cobolt is recognized on the market for providing compact, high performance, continuous wave lasers with reliable single-frequency or narrow-linewidth performance over a large range of environmental conditions. This is thanks to the company’s proprietary HTCure™ technology for advanced laser manufacturing. HTCure™ allows for robust assembly of high precision optical systems into compact, hermetically sealed packages. Cobolt was founded in 2000 and is head-quartered in Solna, Sweden, where the company’s products are developed, designed, and manufactured in a modern and ISO 9001 certified clean-room factory. The facility is specially designed for volume manufacturing of advanced lasers and laser systems. The company has direct sales offices in Sweden, Germany and the USA. Since December 2015, Cobolt AB is part of Hübner Photonics, a division of the Hübner Group. Hübner is a market leading supplier of industrial mobility solutions for the transport industry. Cobolt 04-01, 05-01 and 08-01 Series of high performance CW lasers for Raman spectroscopy 2. What are the different techniques in modern Raman Spectroscopy, and what are they used for? Thanks to rapid technology advancements in recent years, Raman Spectroscopy has become a routine, cost-efficient, and much appreciated analytical tool not only in material research applications but also for in-line process control applications in for instance pharmaceutical, food & beverage, chemical and agricultural industries. -
Raman Amplification
Comunicações Ópticas Prof. H. Salgado Raman amplification Deben Lamon Jelle Stuyvaert Ano lectivo 2007 – 2008 Contents 1 Raman amplification 1 1.1 History . .1 1.2 Stimulated Raman scattering . .1 1.3 Pump and signal equations . .2 1.3.1 Raman gain spectrum . .3 1.3.2 Single-pump Raman amplification . .4 1.3.3 Multiple-pump Raman amplification . .8 1.4 Performance limiting factors . 10 1.4.1 Spontaneous Raman scattering . 10 1.4.2 Rayleigh backscattering . 13 1.4.3 Pump-noise transfer . 14 1.4.4 Polarization mode dispersion . 16 1.5 Ultrafast Raman amplification . 20 2 Distributed Raman amplification 22 2.1 Benefits . 22 2.2 Challenges . 23 2.2.1 Polarization dependence . 23 2.2.2 Spatial overlap of pump and signal mode . 25 2.2.3 Nonlinearities . 26 2.2.4 Rayleigh reflections . 27 2.2.5 Time response . 28 2.3 Backward pumping . 29 2.3.1 Wavelength multiplexed pumps . 30 2.3.2 Broadened pumps . 30 2.3.3 Time-division-multiplexed pumps . 31 2.4 Advanced pumping configurations . 32 2.4.1 Higher order pumping . 32 2.4.2 Quiet pumps . 33 3 Discrete Raman amplification 35 3.1 Basic configuration and model . 35 3.2 Gain fibers and materials . 37 3.3 Design issues . 37 3.3.1 Maximum Raman gain as a function of fiber length . 37 3.3.2 Figure of merit of gain fiber . 37 3.3.3 Efficiency and linearity . 39 3.3.4 Pump-mediated noise . 40 3.3.5 ASE noise figure . 40 3.3.6 Nonlinear effects and double Rayleigh backscattering noise . -
All-Chalcogenide Raman-Parametric Laser, Wavelength Converter and Amplifier in a Single Microwire
All-chalcogenide Raman-parametric Laser, Wavelength Converter and Amplifier in a Single Microwire Raja Ahmad* and Martin Rochette Department of Electrical and Computer Engineering, McGill University, Montreal (QC), Canada, H3A 2A7. PACS Numbers: 42.55.Ye; 42.55.Yj; 42.55.Wd Compact, power efficient and fiber-compatible lasers, wavelength converters and amplifiers are vital ingredients for the future fiber-optic systems and networks. Nonlinear optical effects, like Raman scattering and parametric four-wave mixing, offer a way to realize such devices. Here we use a single chalcogenide microwire to realize a device that provides the functions of a Stokes Raman-parametric laser, a four-wave mixing anti-Stokes wavelength converter, and an ultra-broadband Stokes/anti-Stokes Raman amplifier or supercontinuum generator. The device operation relies on ultrahigh Raman and Kerr gain (upto five orders of magnitude larger than in silica fibers), precisely engineered chromatic dispersion and high photosensitivity of the chalcogenide microwire. The Raman-parametric laser operates at a record low threshold average (peak) pump power of 52 µW (207 mW) and a slope efficiency of >2%. A powerful anti-Stokes signal is generated via the nonlinear four-wave mixing process. As amplifier or the broadband source, the device covers a wavelength (frequency) range of >330 nm (47 THz) when pumped at a wavelength of 1550 nm. Owing to the underlying principle of operation of the device being the nonlinear optical processes, the device is anticipated to operate over the entire transmission window of the chalcogenide glass (λ~1-10 µm). 1 The future of fiber-optic systems lies in the development of all-fiber photonic devices that are compact, power efficient, and can provide novel functionalities. -
Diode-Pumped Multi-Frequency Q-Switched Laser with Intracavity Cascade Raman Emission
Diode-pumped multi-frequency Q-switched laser with intracavity cascade Raman emission Y. T. Chang, Y. P. Huang, K. W. Su, and Y. F. Chen* Department of Electrophysics, National Chiao Tung University, Hsinchu, Taiwan [email protected] Abstract: A diode-pumped actively Q-switched mixed Nd:Y0.3Gd0.7VO4 laser with an intracavity KTP crystal is developed to produce cascade SRS emission up to the fourth order. With an incident pump power of 14 W and a repetition rate of 50 kHz, the average output powers at the first, second , third and fourth Stokes modes are approximately 0.05 W, 0.61 W, 0.25 W, and 0.11 W, respectively. The maximum peak power is greater than 2 kW. ©2008 Optical Society of America OCIS codes: (140.3540) Lasers, Q-switched (140.3550) Lasers, Raman. References and links 1. R. A. Fields, M. Birnbaum, and C. L. Fincher, “Highly efficient Nd:YVO4 diode-laser end-pumped laser,” Appl. Phys. Lett. 51, 1885-1886 (1987). 2. A. Sennaroglu, “Efficient continuous-wave operation of a diode-pumped Nd:YVO4 laser at 1342 nm,” Opt. Commun. 164, 191-197 (1999). 3. Y. F. Chen, “Design criteria for concentration optimization in scaling diode end-pumped lasers to high powers: influence of thermal fracture,” IEEE J. Quantum Electron. 35, 234-239 (1999). 4. A. Y. Yao, W. Hou, Y. P. Kong, L. Guo, L. A. Wu, R. N. Li, D. F. Cui, Z. Y. Xu, Y. Bi, and Y. Zhou, “ Double-end-pumped 11-W Nd:YVO4 cw laser at 1342 nm,” J. -
New Trends in Raman Spectroscopy: from High-Resolution Geochemistry to Planetary Exploration Olivier Beyssac
New Trends in Raman Spectroscopy: From High-Resolution Geochemistry to Planetary Exploration Olivier Beyssac To cite this version: Olivier Beyssac. New Trends in Raman Spectroscopy: From High-Resolution Geochemistry to Plan- etary Exploration. Elements, GeoScienceWorld, 2020. hal-03049557 HAL Id: hal-03049557 https://hal.archives-ouvertes.fr/hal-03049557 Submitted on 9 Dec 2020 HAL is a multi-disciplinary open access L’archive ouverte pluridisciplinaire HAL, est archive for the deposit and dissemination of sci- destinée au dépôt et à la diffusion de documents entific research documents, whether they are pub- scientifiques de niveau recherche, publiés ou non, lished or not. The documents may come from émanant des établissements d’enseignement et de teaching and research institutions in France or recherche français ou étrangers, des laboratoires abroad, or from public or private research centers. publics ou privés. New Trends in Raman Spectroscopy: From High-Resolution Geochemistry to Planetary Exploration Olivier Beyssac1 ABSTRACT This article reviews nonconventional Raman spectroscopy techniques and discusses actual and future applications of these techniques in the Earth and planetary sciences. Time-resolved spectroscopy opens new ways to limit or exploit luminescence effects, whereas techniques based on coherent anti-Stokes Raman scattering or surface- enhanced Raman spectroscopy allow the Raman signal to be considerably enhanced even down to very high spatial resolutions. In addition, compact portable Raman spectrometers are now routinely used out of the laboratory and are even integrated to two rovers going to Mars in the near future. Keywords: mineralogy, SERS/TERS, time-resolved Raman, luminescence, Mars exploration INTRODUCTION Raman spectroscopy is a valuable, commonly-used, technique in the Earth and planetary sciences. -
Efficient Raman Amplifiers and Lasers in Optical Fibers and Silicon
Efficient Raman Amplifiers and Lasers in Optical Fibers and Silicon Waveguides: New Concepts Vom Promotionsausschuss der Technischen Universit¨atHamburg-Harburg zur Erlangung des akademischen Grades Doktor-Ingenieur (Dr.-Ing.) genehmigte Dissertation von Michael Krause aus Hamburg 2007 1. Gutachter: Prof. Dr. Ernst Brinkmeyer, TU Hamburg-Harburg 2. Gutachter: Prof. Dr. Klaus Petermann, TU Berlin 3. Gutachter: Prof. Dr. Klaus Sch¨unemann,TU Hamburg-Harburg Tag der m¨undlichen Pr¨ufung:6. Februar 2007 Uniform Resource Name (URN): urn:nbn:de:gbv:830-tubdok-5769 Danksagung Diese Arbeit ist an der Technischen Universit¨at Hamburg-Harburg w¨ahrend meiner T¨atigkeit als wissenschaftlicher Mitarbeiter in der Arbeitsgruppe Optische Kommu- " nikationstechnik\ entstanden. Ganz herzlich danken m¨ochte ich zun¨achst dem Leiter dieser Arbeitsgruppe, Herrn Prof. Dr. Ernst Brinkmeyer, fur¨ die M¨oglichkeit zur Mitar- beit und Promotion, die umfassende Betreuung und die Unterstutzung¨ s¨amtlicher meiner Vorhaben. Zu gr¨oßtem Dank verpflichtet bin ich weiterhin Hagen Renner fur¨ die unz¨ahligen Gelegenheiten zum ¨außerst ergiebigen fachlichen und nicht-fachlichen Diskutieren und Ideenfinden sowie schließlich fur¨ das Korrekturlesen dieser Arbeit. Bei Sven Cierullies m¨ochte ich mich fur¨ die ergebnisreiche Zusammenarbeit auf dem Gebiet der Raman-Faserlaser und die immer angenehme Stimmung im gemeinsamen Buro¨ bedanken. Weiterhin danke ich Raimonda Stanslovaityte, Robert Draheim, Yi Han und Heiko Fimpel, die im Rahmen ihrer Studien- und Diplomarbeiten hilfreiche Beitr¨age zu meiner Arbeit geliefert haben. Auch allen ubrigen¨ Mitarbeitern und Studenten der Arbeitsgruppe sei gedankt fur¨ ihren Beitrag zu einer gelungenen Arbeitsumgebung; J¨org Voigt danke ich fur¨ die Bera- tung bei praktisch-experimentellen Fragen und Frank Knappe fur¨ vielerlei Hilfestellun- gen. -
An Infrared Spectrometer Utilizing a Spin Flip Raman Laser, IR Frequency Synthesis Techniques, and CO Laser Frequency Standards
1 stss tStfi * I ! I " ^^H^H t"i #£. I ;:>••: *> 19 I ' 'r, s l 3Ef4 0F c ^ c Vv'f o NBS TECHNICAL NOTE 670 U.S. DEPARTMENT OF COMMERCE / National Bureau of Standards An Infrared Spectrometer Utilizing A Spin Flip Raman Laser, IR Frequency Synthesis Techniques, and CO2 Laser Frequency Standards NATIONAL BUREAU OF STANDARDS The National Bureau of Standards' was established by an act of Congress March 3, 1901. The Bureau's overall goal is to strengthen and advance the Nation's science and technology and facilitate their effective application for public benefit. To this end, the Bureau conducts research and provides: (I) a basis for the Nation's physical measurement system, (2) scientific and technological services for industry and government, (3) a technical basis for equity in trade, and (4) technical services to promote public safety. The Bureau consists of the Institute for Basic Standards, the Institute for Materials Research, the Institute tor Applied Technology, the Institute for Computer Sciences and Technology, and the Office for Information Programs. THE INSTITUTE FOR BASIC STANDARDS provides the central basis within the United States of a complete and consistent system of physical measurement; coordinates that system with measurement systems of other nations; and furnishes essential services leading to accurate and uniform physical measurements throughout the Nation's scientific community, industry, and commerce. The Institute consists of the Office of Measurement Services, the Office of Radiation Measurement and the following -
Surface-Enhanced Raman Scattering (SERS)
Technical Note: 51874 Practical Applications of Surface-Enhanced Raman Scattering (SERS) Timothy O. Deschaines, Dick Wieboldt, Thermo Fisher Scientific, Madison, WI, USA Introduction Key Words Surface-Enhanced Raman Scattering (or Spectroscopy), • Amino Acid commonly known as SERS, is a technique that extends the l-alanine range of Raman applications to dilute samples and trace analysis, such as part per million level detection of a • BPE contaminant in water. SERS shows promise in the fields of • Colloidal biochemistry, forensics, food safety, threat detection, and Substrates medical diagnostics. Bacteria on food, trace evidence from a crime scene, cellular materials, or blood glucose are all • Frens Method samples where SERS have been investigated. If SERS is so • Gold Colloids useful, why isn’t it more widespread as an analytical technique? This application note introduces the SERS • L-alanine technique and addresses questions about SERS and how it • Lee and Meisel is applied to Raman. Method What is SERS? more over traditional Raman sampling techniques (some • Raman Laser researchers report an overall 1012 enhancement). An The SERS effect is achieved when an analyte is adsorbed Power important component for a good SERS enhancement is onto or in close proximity to a prepared metal surface. roughness of the metal surface. If the surface is too smooth • SERS The Raman excitation laser produces surface plasmons, or flat there will not be effective surface plasmon (coherent electron oscillations), on the surface of the metal. • Silver Colloids generation. Surface roughness features are usually much These surface plasmons interact with the analyte to greatly smaller than the wavelength of the laser. -
Raman Laser Spectrometer, a Very Demanding Instrument for Planetary Exploration
50th Lunar and Planetary Science Conference 2019 (LPI Contrib. No. 2132) 1519.pdf Raman Laser Spectrometer, a very demanding instrument for planetary exploration J. F. Cabrero* a, M. Fernándezb, M. Colombob, D. Escribanob, P. Gallegob, R. Canchalb, T. Belenguerb, J. García-Martíneza, J. M. Encinasb, L. Bastidea, I. Hutchinsonc, A. Moralb, C.P. Canorab, J. A. R. Prietoa, C. Gordilloa, A. Santiagoa, A. Berrocala, G. Lopez-Reyesd, F. Rulld aIngeniería de Sistemas para la Defensa de España (ISDEFE), C/ Beatriz de Bobadilla 3, 28040, Madrid, Spain. As external contractor of Instituto Nacional de Técnica Aeroespacial (INTA) bINTA, Ctra. Ajalvir, Km 4, 28850, Torrejón de Ardoz, Spain. cDept. of Physics & Astronomy, University of Leicester, University Rd, Leicester, LE1 7RH, UK. dUniversidad de Valladolid - Centro de Astrobiología, Av. Francisco valles, 8, Parque Tecnológico de Boecillo, Parcela 203, E47151 Boecillo, Valladolid, Spain. The Raman Laser Spectrometer (RLS) is one of the Pasteur Payload instruments within the ESA/Roscosmos “ExoMars” 2020 mission. The RLS Instrument is part of the Analytical Laboratory Drawer (ALD), a suite of instruments (together with MicrOmega and MOMA) dedicated to exobiology and geochemistry research, which are accommodated inside of the rover and with the scientific objective of “Searching for evidence of past and present life on Mars". The RLS is a very demanding instrument [1] for searching exobiology in the next planetary missions because its scientific goal consists of perform in-situ Raman spectroscopy over different organic and mineral powder samples of the Mars subsoil after crushing the cores obtained by the rover’s drill system. RLS [2] pretends to characterize the mineral phases produced by water-related processes and to characterize water/geochemical environment as a function of depth in the shallow subsurface.