Download PDF (Inglês)
Total Page:16
File Type:pdf, Size:1020Kb
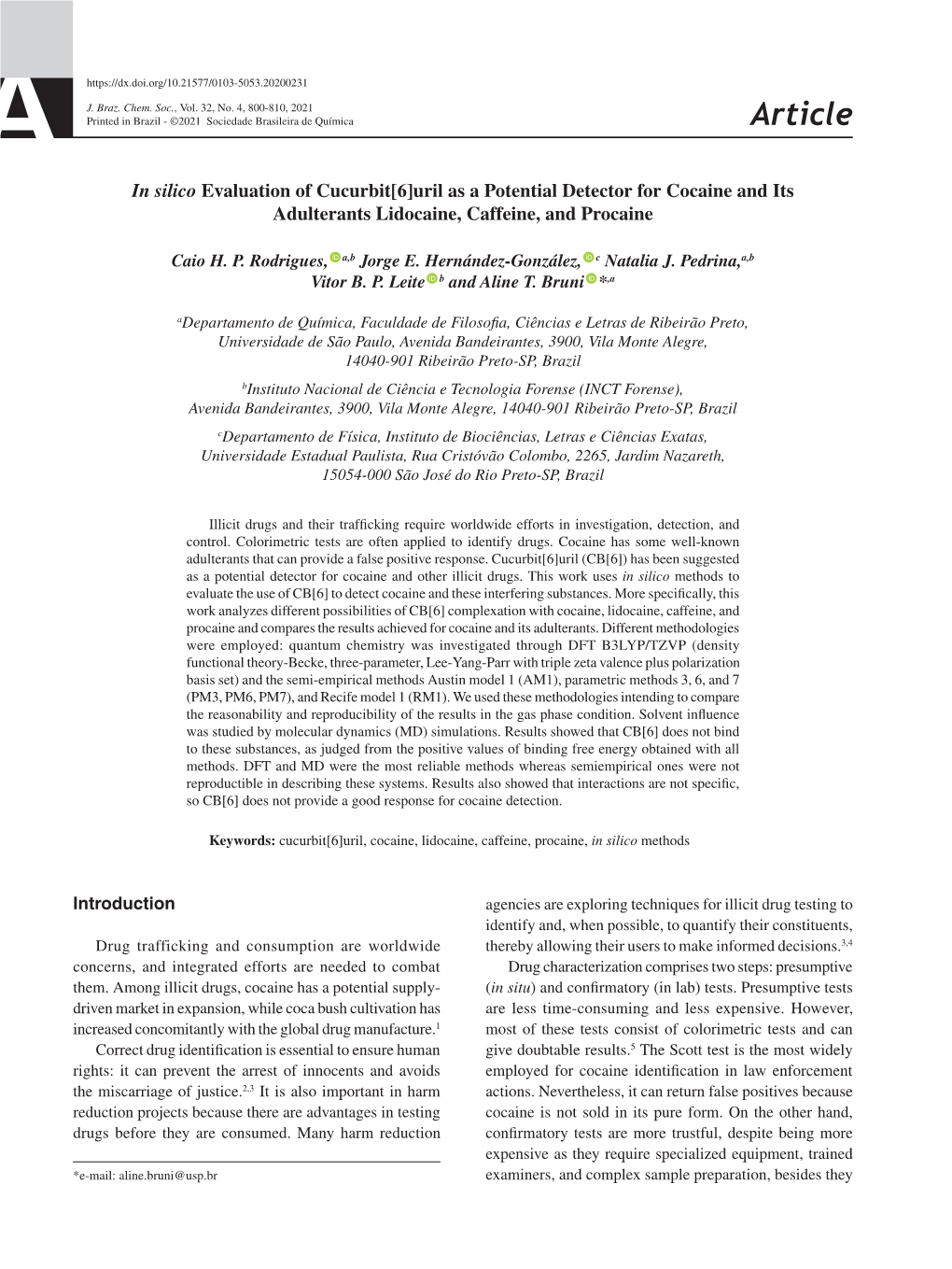
Load more
Recommended publications
-
Semiempirical Implementations of Molecular
Semiempirical Implementations of Molecular Orbital Theory How one can make Hartree-Fock theory less computationally intensive without much sacrificing its accuracy? The most demanding step – calculation of two-electron (four-index) integrals (J and K integrals) appearing in the Fock matrix elements (N4 where N is the number of basis functions). One way to save time – to estimate their value accurately in an a priori fashion and thus to avoid numerical integration. Coulomb integrals measure the repulsion between electrons in regions of space defined by the basis functions. When the basis functions in the integral for one electron are very far from the basis functions for the other, the value of that integral will approach zero. In a large molecule, one might be able to avoid the calculation of a very large number of integrals simply by assuming them to be zero. HF theory is intrinsically inaccurate as it does not include correlation energy. Therefore, modifications of the theory introduced in order to simplify its formalism may actually improve it, provided the new approximations somehow introduce an accounting for correlation energy. Most typically, such approximations involve the adoption of a parametric form for some aspect of the calculation where the parameters involved are chosen so as best reproduce experimental data – ‘semiempirical’. Another motivation for introducing semiempirical approximation into HF theory was to facilitate the computation of derivatives (gradients, Hessians) so that geometries could be more efficiently optimized. Extended Hückel Theory Before considering semiempirical methods we revisit Hückel theory: H11 − ES11 H12 − ES12 L H1N − ES1N H21 − ES21 H22 − ES22 L H2N − ES2N = 0 M M O M H − ES H − ES H − ES N1 N1 N2 N 2 L NN NN The dimension of the secular determinant depends on the choice of the basis set. -
Universit`A Di Parma
UNIVERSITA` DI PARMA DEPARTMENT OF CHEMISTRY, LIFE SCIENCES AND ENVIRONMENTAL SUSTAINABILITY Doctoral Programme in Chemical Sciences XXIX Cycle DYES AND NANOPARTICLES FOR 2PA APPLICATIONS: MODELS AND COMPUTATIONS PhD Student: Somananda Sanyal Supervisors: Prof. Anna Painelli Prof. Swapan K. Pati Coordinator: Prof. Roberto Corradini 2014-2017 Dedicated to My Family List of Abbreviations BFC BF2 complex of Curcumin CT Charge Transfer CNT Carbon Nanotubes D/A Electron Donor / Acceptor 0 DANS p,p -dimethylamino-nitrostilbene DFT Density Functional Theory DMRG Density Matrix Renormalization Group Theory eV electron-volt unit ESM Essential State Model ESP Electrostatic Potential FMO Frontier Molecular Orbital GM G¨oppert-Mayer units (1GM ≡ 10−50 cm 4 s photon−1) HOMO Highest Occupied Molecular Orbital HRS Hyper Rayleigh Scattering LUMO Lowest Unoccupied Molecular Orbital MO Molecular Orbital OPA One Photon Absorption PCM Polarizable Continuum Model PPP Pariser-Parr-Pople TPA Two Photon Absorption ZINDO Zerner's Intermediate Neglect of Differential Overlap i Acknowledgements It's time to say Thank You to many people who have either actively or silently been supporting me in successfully completing the PhD thesis! These three years of my PhD tenure in Parma, Italy has been an eye opener for me, as I slowly learnt to adapt as a girl in a new city. I have been extremely fortunate to meet some people whose influence has been profound and worth cherishing, and I want to grab this opportunity to Thank them All! My parents have been my biggest support system throughout my life and from them I have learnt the first lessons of morality and humanity. -
Biochem Press
Internet Electronic Journal of Molecular Design 2003, 2, 86–95 ISSN 1538–6414 BioChem Press http://www.biochempress.com Internet Electronic Journal of Molecular Design February 2003, Volume 2, Number 2, Pages 86–95 Editor: Ovidiu Ivanciuc Special issue dedicated to Professor Haruo Hosoya on the occasion of the 65th birthday Part 6 Guest Editor: Jun–ichi Aihara Partial Structures of C60 Responsible for Its Lowest Electronic Absorption Band: Corannulene or Triphenylene? Tai–ichi Shibuya,1 Susumu Narita,1 Yasushi Nomura,1 and Tetsuo Morikawa2 1 Department of Chemistry, Faculty of Textile Science and Technology, Shinshu University, Ueda, Nagano–ken, 386–8567, Japan 2 Department of Chemistry, Joetsu University of Education, Joetsu, Niigata–ken, 943–8512, Japan Received: October 21, 2002; Revised: November 22, 2002; Accepted: December 15, 2002; Published: February 28, 2003 Citation of the article: T. Shibuya, S. Narita, Y. Nomura, and T. Morikawa, Partial Structures of C60 Responsible for Its Lowest Electronic Absorption Band: Corannulene or Triphenylene?, Internet Electron. J. Mol. Des. 2003, 2, 86–95, http://www.biochempress.com. Copyright © 2003 BioChem Press T. Shibuya, S. Narita, Y. Nomura, and T. Morikawa Internet Electronic Journal of Molecular Design 2003, 2, 86–95 Internet Electronic Journal BioChem Press of Molecular Design http://www.biochempress.com Partial Structures of C60 Responsible for Its Lowest Electronic Absorption Band: Corannulene or Triphenylene?# Tai–ichi Shibuya,1,* Susumu Narita,1 Yasushi Nomura,1 and Tetsuo Morikawa2 1 Department of Chemistry, Faculty of Textile Science and Technology, Shinshu University, Ueda, Nagano–ken, 386–8567, Japan 2 Department of Chemistry, Joetsu University of Education, Joetsu, Niigata–ken, 943–8512, Japan Received: October 21, 2002; Revised: November 22, 2002; Accepted: December 15, 2002; Published: February 28, 2003 Internet Electron. -
Α-Acetylpyridine Ketone'un Ve Argerol'lü Bileşiğinin
α-ACETYLPYRIDINE KETONE’UN VE ARGEROL'LÜ BİLEŞİĞİNİN TİTREŞİMLERİNİN DENEYSEL VE TEORİK OLARAK İNCELENMESİ ABDULLAH ATILGAN YÜKSEK LİSANS TEZİ FİZİK GAZİ ÜNİVERSİTESİ FEN BİLİMLERİ ENSTİTÜSÜ ŞUBAT 2013 ANKARA Abdullah ATILGAN tarafından hazırlanan “ α - ACETYLPYRIDINE KETONE’UN VE ARGEROL'LÜ BİLEŞİĞİNİN TİTREŞİMLERİNİN DENEYSEL VE TEORİK OLARAK İNCELENMESİ” adlı bu tezin Yüksek Lisans tezi olarak uygun olduğunu onaylarım. Prof. Dr. Şenay YURDAKUL ………………………………….. Tez Danışmanı, Fizik Anabilim Dalı Bu çalışma, jürimiz tarafından oy birliği ile Fizik Anabilim Dalında Yüksek Lisans tezi olarak kabul edilmiştir. Prof. Dr. Atike Semra BİLGİÇ ………………………………….. Kimya Anabilim Dalı, Ankara Üniversitesi Prof. Dr. Şenay YURDAKUL ………………………………….. Fizik Anabilim Dalı, Gazi Üniversitesi Doç. Dr. Semran SAĞLAM ………………………………….. Fizik Anabilim Dalı, Gazi Üniversitesi Tez SavunmaTarihi: 04/02/2013 Bu tez ile G.Ü. Fen Bilimleri Enstitüsü Yönetim Kurulu Yüksek Lisans derecesini onamıştır. Prof.Dr. Şeref SAĞIROĞLU ………………………………….. Fen Bilimleri Enstitüsü Müdürü TEZ BİLDİRİMİ Tez içindeki bütün bilgilerin etik davranış ve akademik kurallar çerçevesinde elde edilerek sunulduğunu, ayrıca tez yazım kurallarına uygun olarak hazırlanan bu çalışmada bana ait olmayan her türlü kaynağa eksiksiz atıf yapıldığını bildiririm. ABDULLAH ATILGAN iv α-ACETYLPYRİDİNE KETONE’ÜN VE ARGEROL'LÜ BİLEŞİĞİNİN TİTREŞİMLERİNİN DENEYSEL VE TEORİK OLARAK İNCELENMESİ (Yüksek Lisans Tezi) Abdullah ATILGAN GAZİ ÜNİVERSİTESİ FEN BİLİMLERİ ENSTİTÜSÜ Şubat 2013 ÖZET Bu çalışmada C7H7NO (α-acetylpyridin ketone) genel formülü ile verilen ligandın ve argerollü bileşiğinin IR spektrumu kaydedilip bu bileşiklerin titreşim frekans ve kipleri saptandı. Ligandın ve bileşiğin titreşim frekanslarına işaretleme yapıldı. Gaussian 09 bilgisayar programı yardımıyla teorik frekans değerleri, geometrik parametreler ve NBO yük dağılımı elde edildi ve bu değerler deneysel sonuçlar ile karşılaştırıldı. Bilim Kodu : 202.1.008 Anahtar Kelimeler : α-acetylpyridine ketone, argerol bileşiği, IR, Ra, NBO Sayfa Adedi : 65 Tez Yöneticisi : Prof. -
Computational Chemistry: a Practical Guide for Applying Techniques to Real-World Problems
Computational Chemistry: A Practical Guide for Applying Techniques to Real-World Problems. David C. Young Copyright ( 2001 John Wiley & Sons, Inc. ISBNs: 0-471-33368-9 (Hardback); 0-471-22065-5 (Electronic) COMPUTATIONAL CHEMISTRY COMPUTATIONAL CHEMISTRY A Practical Guide for Applying Techniques to Real-World Problems David C. Young Cytoclonal Pharmaceutics Inc. A JOHN WILEY & SONS, INC., PUBLICATION New York . Chichester . Weinheim . Brisbane . Singapore . Toronto Designations used by companies to distinguish their products are often claimed as trademarks. In all instances where John Wiley & Sons, Inc., is aware of a claim, the product names appear in initial capital or all capital letters. Readers, however, should contact the appropriate companies for more complete information regarding trademarks and registration. Copyright ( 2001 by John Wiley & Sons, Inc. All rights reserved. No part of this publication may be reproduced, stored in a retrieval system or transmitted in any form or by any means, electronic or mechanical, including uploading, downloading, printing, decompiling, recording or otherwise, except as permitted under Sections 107 or 108 of the 1976 United States Copyright Act, without the prior written permission of the Publisher. Requests to the Publisher for permission should be addressed to the Permissions Department, John Wiley & Sons, Inc., 605 Third Avenue, New York, NY 10158-0012, (212) 850-6011, fax (212) 850-6008, E-Mail: PERMREQ @ WILEY.COM. This publication is designed to provide accurate and authoritative information in regard to the subject matter covered. It is sold with the understanding that the publisher is not engaged in rendering professional services. If professional advice or other expert assistance is required, the services of a competent professional person should be sought. -
UFRRJ Catarina De Nigris Del Cistia
UFRRJ INSTITUTO DE CIÊNCIAS EXATAS PROGRAMA DE PÓS-GRADUAÇÃO EM QUÍMICA TESE MODELAGEM MOLECULAR APLICADA AO ESTUDO DE REAÇÕES DE INIBIÇÃO ENZIMÁTICA COM APLICAÇÃO POTENCIAL NO CONTROLE DE Leishmania amazonensis Catarina De Nigris Del Cistia 2010 Livros Grátis http://www.livrosgratis.com.br Milhares de livros grátis para download. UNIVERSIDADE FEDERAL RURAL DO RIO DE JANEIRO INSTITUTO DE CIÊNCIAS EXATAS PROGRAMA DE PÓS-GRADUAÇÃO EM QUÍMICA MODELAGEM MOLECULAR APLICADA AO ESTUDO DE REAÇÕES DE INIBIÇÃO ENZIMÁTICA COM APLICAÇÃO POTENCIAL NO CONTROLE DE Leishmania amazonensis CATARINA DE NIGRIS DEL CISTIA Sob a orientação do Professor Carlos Maurício Rabello de Sant’Anna e Co-orientação da Professora Aurea Echevarria Tese submetida como requisito parcial para obtenção do grau de Doutor em Ciências, no Programa de Pós- Graduação em Química, Área de Concentração em Química Orgânica Seropédica, RJ Junho de 2010 UFRRJ / Biblioteca Central / Divisão de Processamentos Técnicos 547.21 D331m Del Cistia, Catarina de Nigris, 1980-. T Modelagem molecular aplicada ao estudo de reações de inibição enzimática com aplicação potencial no controle de Leishmania amazonensis / Catarina de Nigris Del Cistia – 2010. 193 f.: il. Orientador: Carlos Maurício Rabello de Sant’Anna. Tese (doutorado) – Universidade Federal Rural do Rio de Janeiro, Programa de Pós- Graduação em Química. Bibliografia: f. 144-159. 1. Síntese orgânica – Teses. 2. Leishmania - Teses. 3. Leishmaniose - Teses. 4. Inibidores enzimáticos – Teses. I. Sant’Anna, Carlos Maurício Rabello de, 1965-. II. Universidade Federal Rural do Rio de Janeiro. Programa de Pós-Graduação em Química. III. Título. “Os loucos abrem os caminhos que mais tarde os sábios percorrem.” James Joyce AGRADECIMENTOS . -
11. MOLECULAR MODELING Chemists Frequently
11. MOLECULAR MODELING Chemists frequently use models to better understand chemical phenomena. In this laboratory models are used to analyze chemical bonding and molecular structure. We will start with the Lewis electron dot (Localized Electron) model1 and use it to predict the electronic structure of some simple molecules. We will then use ball-and-stick models to construct three-dimensional models of those molecules. The three-dimensional models will be refined using Gillespie’s Valence Shell Electron Pair Repulsion (VSEPR) model2. Finally, we will use Spartan3, a computational chemistry program, to calculate the molecular geometry. The results from Spartan will then be compared with the VSEPR results. Computational chemistry. In theory, all of chemistry can be understood as solutions of Schrödinger’s wave equation. In practice, solution of the Schrödinger equation is so complicated that chemically accurate calculations for molecules involving more than three electrons and three nuclei, e.g. H3, are beyond the reach of current computer hardware/algorithms. To model the properties of larger (and more interesting) molecules, chemists must use some form of approximate computational method. The simplest, and thus fastest, computational method is called molecular mechanics. In molecular mechanics the bonds of a molecule are treated as springs and the force constants of the springs are determined from experimental measurements. The overall molecular energy is the sum of the energies of stretching, bending and torsion (the molecular force field) for all of the bonds in the molecule. Although molecular mechanics ignores electronic properties, it gives quite good structural results for large molecules such as proteins. Drug companies performed much of the pioneering work on molecular mechanics. -
Fundamentals of Quantum Mechanics for Chemistry
Fundamentals of Quantum Mechanics for Chemistry G´eraldMONARD Equipe de Chimie et Biochimie Th´eoriques UMR 7565 CNRS - Universit´eHenri Poincar´e Facult´edes Sciences - B.P. 239 54506 Vandœuvre-les-Nancy Cedex - FRANCE http://www.monard.info/ Outline . 1. Fundamentals of Quantum Mechanics for Chemistry 3 Hartree-Fock methods 3 Density Functional Theory 3 The QM scaling problem 3 Semiempirical methods 3 Molecular Mechanics 2. Fundamentals of QM/MM methods 3 Partionning 3 QM/MM interactions 3 Cutting covalent bonds 3 ONIOM 3 Some available software 3. Selected QM/MM applications 3 Solvent effects 3 Spectroscopy 3 Biochemistry . Outline 4. Fundamentals of Linear Scaling methods 3 QM Bottlenecks 3 General ideas and solutions 3 Some available software 5. Focus on some Linear Scaling methods 3 CG-DMS 3 Mozyme 3 Divide & Conquer 6. Selected Linear Scaling applications 3 Energy Decomposition; Charge Transfer & Polarization 3 Born-Oppenheimer Molecular Dynamics 7. Parallelization of QM/MM and Linear Scaling methods Fundamentals of Quantum Mechanics for Chemistry (1) Some approximations And there was the Schr¨odinger equation. H0Ψ0 = E0Ψ0 where: H0 is an Hamiltonian operator that describes a molecular system Ψ0 is a wavefunction (solution of the Schr¨odingerequation) that describe a state of the system E0 the energy associated to Ψ0 + 1 equation + 2 unknowns (given H0) = +¥ solutions! From now on: 3 ground state 3 closed shell 3 non-relativistic Fundamentals of Quantum Mechanics for Chemistry (2) Born-Oppenheimer approximation nuclei are fixed point charges only electrons are represented by a wavefunction Ψ HΨ = Eelec Ψ (1) H = Te + VeN + Vee 1 −Z 1 K (2) = − ∑∆i + ∑∑ + ∑∑ 2 i i K riK i i>j rij | {z } | {z } | {z } kinetic energy e−-nuclei inter. -
Semiemprical Methods Chapter 5 Semiemprical Methods
Semiemprical Methods Chapter 5 Semiemprical Methods Because of the difficulties in applying ab initio methods to medium and large molecules, many semiempirical methods have been developed to treat such molecules. The earliest semiempirical methods treated only the π electrons of conjugated molecules. In the π-electron approximation, the nπ π electrons are treated separately by incorporating the effects of the σ electrons and the nuclei into some sort of effective π- electron Hamiltonian Ĥπ (recall the similar valence-electron approximation where Vi is the potential energy of the ith π electron in the field produced by the nuclei and the σ electrons. The core is everything except the π electrons. The most celebrated semiempirical π-electron theory is the Hückel molecular-orbital (HMO) method, developed in the 1930s to treat planar conjugated hydrocarbons. Here the π-electron Hamiltonian is approximated by the simpler form where Ĥeff(i) incorporates the effects of the π-electron repulsions in an average way. In fact the Hückel method does not specify any explicit form for Ĥeff(i). Since the Hückel π-electron Hamiltonian is the sum of one-electron Hamiltonians, a separation of variables is possible. We have The wave function takes no account of spin or the antisymmetry requirement. To do so, we must put each electron in a spin-orbital ui=ii. The wave function π is then written as a Slater determinant of spin-orbitals. Since Ĥeff(i) is not specified, there is no point in trying to solve above eq. directly. Instead, the variational method is used. The next assumption in the HMO method is to approximate the π MOs as LCAOs. -
Semiempirical Methods
Chapter 7 Semiempirical Methods 7.1 Introduction Semiempirical methods modify Hartree-Fock (HF) calculations by introducing functions with empirical parameters. These parameters are adjusted with experimen- tal conclusions to improve the quality of computation. The real cost of computation is due to the two-electron integrals in the Hamiltonian that has been simplified in this method. Semiempirical methods are based on three approximation schemes. 1. The elimination of the core electrons from the calculation. Inner electrons do not contribute towards chemical activity, which makes it pos- sible to remove the core electron functions from the Hamiltonian calculation. Normally, the entire core (the nucleus and core electrons) of atoms is replaced by a parameterized function. This has the effect of drastically reducing the com- plexity of the calculation without a major impact on the accuracy. 2. The use of the minimum number of basis sets. In this approximation, while introducing the functions of valence electrons, only the minimum required number of basis sets will be used. This technique also reduces the complexity of computation to a large extent. 3. The reduction of the number of two-electron integrals. This approximation is introduced on the basis of experimentation rather than chemical grounds. The majority of the work in ab initio calculations is in the evaluation of the two electron integrals (Coulomb and exchange). All modern semiempirical methods are based on the modified neglect of differential over- lap (MNDO) approach. In this method, parameters are assigned for different atomic types and are fitted to reproduce properties such as heats of formation, geometrical variables, dipole moments, and first ionization energies. -
UFRRJ Catarina De Nigris Del Cistia
UFRRJ INSTITUTO DE CIÊNCIAS EXATAS PROGRAMA DE PÓS-GRADUAÇÃO EM QUÍMICA TESE MODELAGEM MOLECULAR APLICADA AO ESTUDO DE REAÇÕES DE INIBIÇÃO ENZIMÁTICA COM APLICAÇÃO POTENCIAL NO CONTROLE DE Leishmania amazonensis Catarina De Nigris Del Cistia 2010 UNIVERSIDADE FEDERAL RURAL DO RIO DE JANEIRO INSTITUTO DE CIÊNCIAS EXATAS PROGRAMA DE PÓS-GRADUAÇÃO EM QUÍMICA MODELAGEM MOLECULAR APLICADA AO ESTUDO DE REAÇÕES DE INIBIÇÃO ENZIMÁTICA COM APLICAÇÃO POTENCIAL NO CONTROLE DE Leishmania amazonensis CATARINA DE NIGRIS DEL CISTIA Sob a orientação do Professor Carlos Maurício Rabello de Sant’Anna e Co-orientação da Professora Aurea Echevarria Tese submetida como requisito parcial para obtenção do grau de Doutor em Ciências, no Programa de Pós- Graduação em Química, Área de Concentração em Química Orgânica Seropédica, RJ Junho de 2010 UFRRJ / Biblioteca Central / Divisão de Processamentos Técnicos 547.21 D331m Del Cistia, Catarina de Nigris, 1980-. T Modelagem molecular aplicada ao estudo de reações de inibição enzimática com aplicação potencial no controle de Leishmania amazonensis / Catarina de Nigris Del Cistia – 2010. 193 f.: il. Orientador: Carlos Maurício Rabello de Sant’Anna. Tese (doutorado) – Universidade Federal Rural do Rio de Janeiro, Programa de Pós- Graduação em Química. Bibliografia: f. 144-159. 1. Síntese orgânica – Teses. 2. Leishmania - Teses. 3. Leishmaniose - Teses. 4. Inibidores enzimáticos – Teses. I. Sant’Anna, Carlos Maurício Rabello de, 1965-. II. Universidade Federal Rural do Rio de Janeiro. Programa de Pós-Graduação em Química. III. Título. “Os loucos abrem os caminhos que mais tarde os sábios percorrem.” James Joyce AGRADECIMENTOS . Aos meus pais Ana Maria De Nigris e Roberto Diniz Del Cistia, e aos irmãos Roberto e Guilherme De Nigris Del Cistia, pelo amor, paciência, apoio e carinho durante toda esta jornada. -
Firefly 8.0.0 Manual
Firefly 8.0.0 manual Version 2013-11-12 Table of Contents Introduction About this manual Overview of capabilities Firefly and GAMESS (US) Release history Citing Firefly Installing and running Firefly General information Windows Windows MPI implementations Windows and CUDA Linux Linux MPI implementations Linux and CUDA Installing Firefly on 64-bit Linux cluster with InfiniBand network and Intel MPI Command line options Creating an input file Input file structure Chemical control data Computer related control data Formatted input sections Input checking Input preprocessing Performance Introduction 64-bit processing support The P2P communication interface The XP and extended XP parallel modes of execution Utilizing HyperThreading CUDA The Fastdiag dynamic library Fast two-electron integrals code Quantum fast multipole method Output Main output The PUNCH file The IRCDATA file The MCQD files - 1 - Restart capabilities Coordinate types Introduction Cartesian coordinates Z-Matrix and natural internal coordinates Delocalized coordinates Utilizing symmetry Isotopic substitution Basis sets Introduction Built-in basis sets Using pure spherical polarization functions Using an external basis set file Manually specifying a basis set Using effective core potentials Partial linear dependence in a basis set Basis set superposition error (BSSE) correction Starting orbitals General accuracy switches Semi-empirical methods Introduction Available methods Hartree-Fock theory Introduction Restricted Hartree-Fock Unrestricted Hartree-Fock Restricted-open Hartree-Fock