Mitochondrial Membrane Transporters and Metabolic Switch in Heart Failure
Total Page:16
File Type:pdf, Size:1020Kb
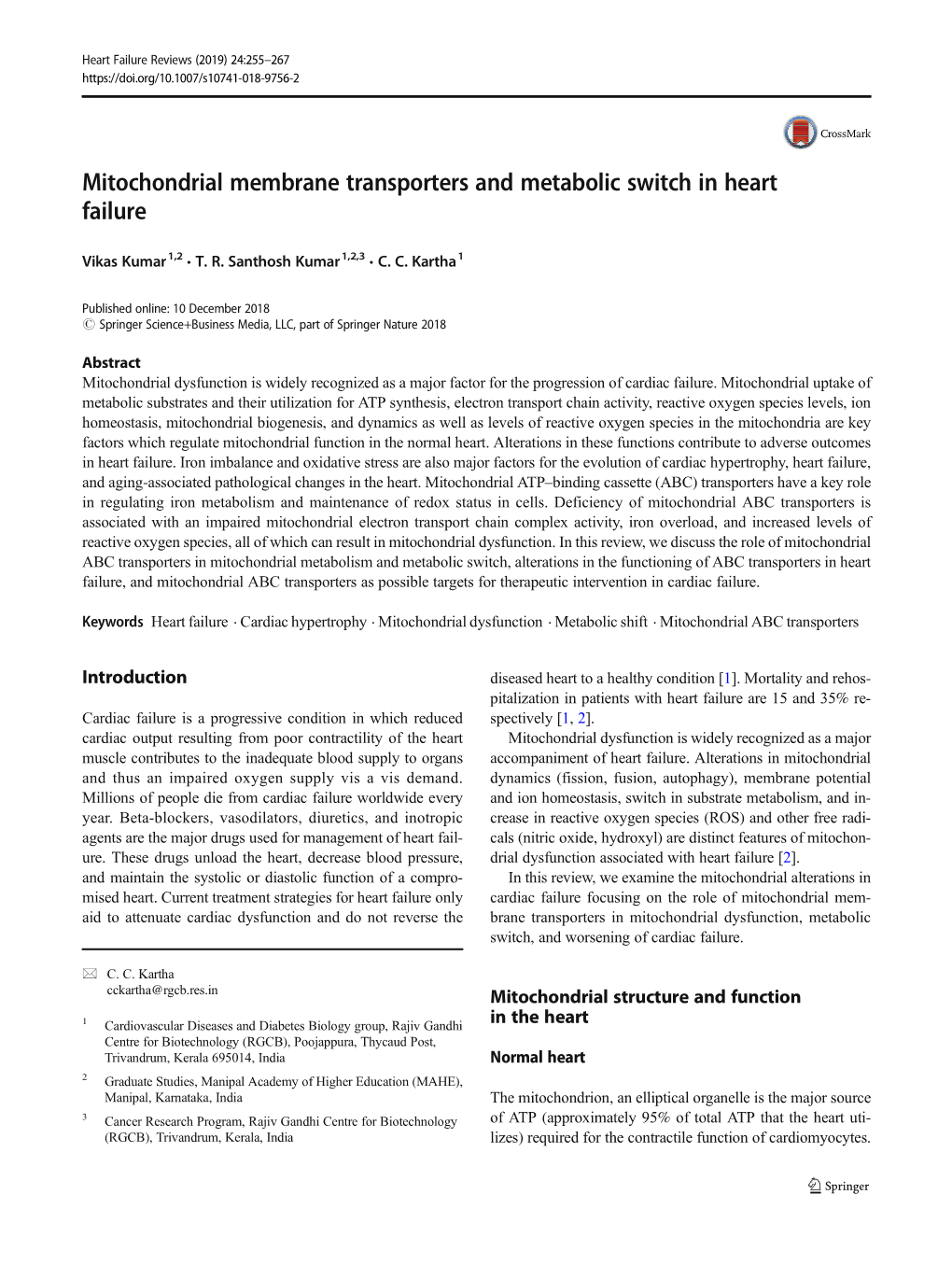
Load more
Recommended publications
-
Iron Depletion Reduces Abce1 Transcripts While Inducing The
Preprints (www.preprints.org) | NOT PEER-REVIEWED | Posted: 22 October 2019 doi:10.20944/preprints201910.0252.v1 1 Research Article 2 Iron depletion Reduces Abce1 Transcripts While 3 Inducing the Mitophagy Factors Pink1 and Parkin 4 Jana Key 1,2, Nesli Ece Sen 1, Aleksandar Arsovic 1, Stella Krämer 1, Robert Hülse 1, Suzana 5 Gispert-Sanchez 1 and Georg Auburger 1,* 6 1 Experimental Neurology, Goethe University Medical School, 60590 Frankfurt am Main; 7 2 Faculty of Biosciences, Goethe-University Frankfurt am Main, Germany 8 * Correspondence: [email protected] 9 10 Abstract: Lifespan extension was recently achieved in Caenorhabditis elegans nematodes by 11 mitochondrial stress and mitophagy, triggered via iron depletion. Conversely in man, deficient 12 mitophagy due to Pink1/Parkin mutations triggers iron accumulation in patient brain and limits 13 survival. We now aimed to identify murine fibroblast factors, which adapt their mRNA expression 14 to acute iron manipulation, relate to mitochondrial dysfunction and may influence survival. After 15 iron depletion, expression of the plasma membrane receptor Tfrc with its activator Ireb2, the 16 mitochondrial membrane transporter Abcb10, the heme-release factor Pgrmc1, the heme- 17 degradation enzyme Hmox1, the heme-binding cholesterol metabolizer Cyp46a1, as well as the 18 mitophagy regulators Pink1 and Parkin showed a negative correlation to iron levels. After iron 19 overload, these factors did not change expression. Conversely, a positive correlation of mRNA levels 20 with both conditions of iron availability was observed for the endosomal factors Slc11a2 and Steap2, 21 as well as for the iron-sulfur-cluster (ISC)-containing factors Ppat, Bdh2 and Nthl1. -
Protein Identities in Evs Isolated from U87-MG GBM Cells As Determined by NG LC-MS/MS
Protein identities in EVs isolated from U87-MG GBM cells as determined by NG LC-MS/MS. No. Accession Description Σ Coverage Σ# Proteins Σ# Unique Peptides Σ# Peptides Σ# PSMs # AAs MW [kDa] calc. pI 1 A8MS94 Putative golgin subfamily A member 2-like protein 5 OS=Homo sapiens PE=5 SV=2 - [GG2L5_HUMAN] 100 1 1 7 88 110 12,03704523 5,681152344 2 P60660 Myosin light polypeptide 6 OS=Homo sapiens GN=MYL6 PE=1 SV=2 - [MYL6_HUMAN] 100 3 5 17 173 151 16,91913397 4,652832031 3 Q6ZYL4 General transcription factor IIH subunit 5 OS=Homo sapiens GN=GTF2H5 PE=1 SV=1 - [TF2H5_HUMAN] 98,59 1 1 4 13 71 8,048185945 4,652832031 4 P60709 Actin, cytoplasmic 1 OS=Homo sapiens GN=ACTB PE=1 SV=1 - [ACTB_HUMAN] 97,6 5 5 35 917 375 41,70973209 5,478027344 5 P13489 Ribonuclease inhibitor OS=Homo sapiens GN=RNH1 PE=1 SV=2 - [RINI_HUMAN] 96,75 1 12 37 173 461 49,94108966 4,817871094 6 P09382 Galectin-1 OS=Homo sapiens GN=LGALS1 PE=1 SV=2 - [LEG1_HUMAN] 96,3 1 7 14 283 135 14,70620005 5,503417969 7 P60174 Triosephosphate isomerase OS=Homo sapiens GN=TPI1 PE=1 SV=3 - [TPIS_HUMAN] 95,1 3 16 25 375 286 30,77169764 5,922363281 8 P04406 Glyceraldehyde-3-phosphate dehydrogenase OS=Homo sapiens GN=GAPDH PE=1 SV=3 - [G3P_HUMAN] 94,63 2 13 31 509 335 36,03039959 8,455566406 9 Q15185 Prostaglandin E synthase 3 OS=Homo sapiens GN=PTGES3 PE=1 SV=1 - [TEBP_HUMAN] 93,13 1 5 12 74 160 18,68541938 4,538574219 10 P09417 Dihydropteridine reductase OS=Homo sapiens GN=QDPR PE=1 SV=2 - [DHPR_HUMAN] 93,03 1 1 17 69 244 25,77302971 7,371582031 11 P01911 HLA class II histocompatibility antigen, -
ABCB7 Gene ATP Binding Cassette Subfamily B Member 7
ABCB7 gene ATP binding cassette subfamily B member 7 Normal Function The ABCB7 gene provides instructions for making a protein known as an ATP-binding cassette (ABC) transporter. ABC transporter proteins carry many types of molecules across membranes in cells. The ABCB7 protein is located in the inner membrane of cell structures called mitochondria. Mitochondria are involved in a wide variety of cellular activities, including energy production, chemical signaling, and regulation of cell growth and division. In the mitochondria of developing red blood cells (erythroblasts), the ABCB7 protein plays a critical role in the production of heme. Heme contains iron and is a component of hemoglobin, the protein that carries oxygen in the blood. The ABCB7 protein is also involved in the formation of certain proteins containing clusters of iron and sulfur atoms (Fe-S clusters). Researchers suspect that the ABCB7 protein transports Fe-S clusters from mitochondria, where they are formed, to the surrounding cellular fluid (cytosol), where they can be incorporated into proteins. Overall, researchers believe that the ABCB7 protein helps maintain an appropriate balance of iron (iron homeostasis) in developing red blood cells. Health Conditions Related to Genetic Changes X-linked sideroblastic anemia and ataxia At least three mutations in the ABCB7 gene have been identified in people with X-linked sideroblastic anemia with ataxia. Each of these mutations changes a single protein building block (amino acid) in the ABCB7 protein, slightly altering its structure. These changes disrupt the protein's usual role in heme production and iron homeostasis. Anemia results when heme cannot be produced normally, and therefore not enough hemoglobin is made. -
Structures and Functions of Mitochondrial ABC Transporters
ATP-binding cassette transporters: from mechanism to organism 943 Structures and functions of mitochondrial ABC transporters Theresia A. Schaedler*, Belinda Faust†, Chitra A. Shintre†, Elisabeth P. Carpenter†, Vasundara Srinivasan‡, Hendrik W. van Veen§ and Janneke Balk1 *Department of Biological Chemistry and Crop Protection, Rothamsted Research, West Common, Harpenden, AL5 2JQ, U.K. †Structural Genomics Consortium, Nuffield Department of Clinical Medicine, University of Oxford, Oxford, OX3 7DQ, U.K. ‡LOEWE center for synthetic microbiology (SYNMIKRO) and Philipps University, D-35043 Marburg, Germany §Department of Pharmacology, University of Cambridge, Tennis Court Road, Cambridge, CB2 1PD, U.K. John Innes Centre and University of East Anglia, Colney Lane, Norwich, NR4 7UH, U.K. Abstract A small number of physiologically important ATP-binding cassette (ABC) transporters are found in mitochondria. Most are half transporters of the B group forming homodimers and their topology suggests they function as exporters. The results of mutant studies point towards involvement in iron cofactor biosynthesis. In particular, ABC subfamily B member 7 (ABCB7) and its homologues in yeast and plants are required for iron-sulfur (Fe-S) cluster biosynthesis outside of the mitochondria, whereas ABCB10 is involved in haem biosynthesis. They also play a role in preventing oxidative stress. Mutations in ABCB6 and ABCB7 have been linked to human disease. Recent crystal structures of yeast Atm1 and human ABCB10 have been key to identifying substrate-binding sites and transport mechanisms. Combined with in vitro and in vivo studies, progress is being made to find the physiological substrates of the different mitochondrial ABC transporters. Sequence analysis of mitochondrial ABC The ABCB7 group, which includes the ABC transporters transporters of the mitochondria Atm1 in yeast and ATM3 in Arabidopsis, Mitochondria of most eukaryote species harbour 2–4 can be found in virtually all eukaryotic species. -
ABCB6 Is a Porphyrin Transporter with a Novel Trafficking Signal That Is Conserved in Other ABC Transporters Yu Fukuda University of Tennessee Health Science Center
University of Tennessee Health Science Center UTHSC Digital Commons Theses and Dissertations (ETD) College of Graduate Health Sciences 12-2008 ABCB6 Is a Porphyrin Transporter with a Novel Trafficking Signal That Is Conserved in Other ABC Transporters Yu Fukuda University of Tennessee Health Science Center Follow this and additional works at: https://dc.uthsc.edu/dissertations Part of the Chemicals and Drugs Commons, and the Medical Sciences Commons Recommended Citation Fukuda, Yu , "ABCB6 Is a Porphyrin Transporter with a Novel Trafficking Signal That Is Conserved in Other ABC Transporters" (2008). Theses and Dissertations (ETD). Paper 345. http://dx.doi.org/10.21007/etd.cghs.2008.0100. This Dissertation is brought to you for free and open access by the College of Graduate Health Sciences at UTHSC Digital Commons. It has been accepted for inclusion in Theses and Dissertations (ETD) by an authorized administrator of UTHSC Digital Commons. For more information, please contact [email protected]. ABCB6 Is a Porphyrin Transporter with a Novel Trafficking Signal That Is Conserved in Other ABC Transporters Document Type Dissertation Degree Name Doctor of Philosophy (PhD) Program Interdisciplinary Program Research Advisor John D. Schuetz, Ph.D. Committee Linda Hendershot, Ph.D. James I. Morgan, Ph.D. Anjaparavanda P. Naren, Ph.D. Jie Zheng, Ph.D. DOI 10.21007/etd.cghs.2008.0100 This dissertation is available at UTHSC Digital Commons: https://dc.uthsc.edu/dissertations/345 ABCB6 IS A PORPHYRIN TRANSPORTER WITH A NOVEL TRAFFICKING SIGNAL THAT -
Genome-Wide Identification of Whole ATP-Binding Cassette (ABC)
Jeong et al. BMC Genomics 2014, 15:651 http://www.biomedcentral.com/1471-2164/15/651 RESEARCH ARTICLE Open Access Genome-wide identification of whole ATP-binding cassette (ABC) transporters in the intertidal copepod Tigriopus japonicus Chang-Bum Jeong1, Bo-Mi Kim2, Jae-Seong Lee2* and Jae-Sung Rhee3* Abstract Backgrounds: The ATP-binding cassette (ABC) transporter superfamily is one of the largest transporter gene families and is observed in all animal taxa. Although a large set of transcriptomic data was recently assembled for several species of crustaceans, identification and annotation of the large ABC transporter gene family have been very challenging. Results: In the intertidal copepod Tigriopus japonicus, 46 putative ABC transporters were identified using in silico analysis, and their full-length cDNA sequences were characterized. Phylogenetic analysis revealed that the 46 T. japonicus ABC transporters are classified into eight subfamilies (A-H) that include all the members of all ABC subfamilies, consisting of five ABCA, five ABCB, 17 ABCC, three ABCD, one ABCE, three ABCF, seven ABCG, and five ABCH subfamilies. Of them, unique isotypic expansion of two clades of ABCC1 proteins was observed. Real-time RT-PCR-based heatmap analysis revealed that most T. japonicus ABC genes showed temporal transcriptional expression during copepod development. The overall transcriptional profile demonstrated that half of all T. japonicus ABC genes were strongly associated with at least one developmental stage. Of them, transcripts TJ-ABCH_88708 and TJ-ABCE1 were highly expressed during all developmental stages. Conclusions: The whole set of T. japonicus ABC genes and their phylogenetic relationships will provide a better understanding of the comparative evolution of essential gene family resources in arthropods, including the crustacean copepods. -
ABCD3 (F-1): Sc-514728
SANTA CRUZ BIOTECHNOLOGY, INC. ABCD3 (F-1): sc-514728 BACKGROUND APPLICATIONS The peroxisomal membrane contains several ATP-binding cassette (ABC) ABCD3 (F-1) is recommended for detection of ABCD3 of human origin by transporters, ABCD1-4 that are known to be present in the human peroxisome Western Blotting (starting dilution 1:100, dilution range 1:100-1:1000), membrane. All four proteins are ABC half-transporters, which dimerize to form immunoprecipitation [1-2 µg per 100-500 µg of total protein (1 ml of cell an active transporter. A mutation in the ABCD1 gene causes X-linked adreno- lysate)], immunofluorescence (starting dilution 1:50, dilution range 1:50- leukodystrophy (X-ALD), a peroxisomal disorder which affects lipid storage. 1:500) and solid phase ELISA (starting dilution 1:30, dilution range 1:30- ABCD2 in mouse is expressed at high levels in the brain and adrenal organs, 1:3000). which are adversely affected in X-ALD. The peroxisomal membrane comprises Suitable for use as control antibody for ABCD3 siRNA (h): sc-41147, ABCD3 two quantitatively major proteins, PMP22 and ABCD3. ABCD3 is associated shRNA Plasmid (h): sc-41147-SH and ABCD3 shRNA (h) Lentiviral Particles: with irregularly shaped vesicles which may be defective peroxisomes or per- sc-41147-V. oxisome precursors. ABCD1 localizes to peroxisomes. ABCB7 is a half-trans- porter involved in the transport of heme from the mitochondria to the cytosol. Molecular Weight of ABCD3: 75 kDa. Positive Controls: HeLa whole cell lysate: sc-2200, SH-SY5Y cell lysate: REFERENCES sc-3812 or Caco-2 cell lysate: sc-2262. -
Three Hundred Twenty-Six Genetic Variations in Genes Encoding Nine Members of ATP-Binding Cassette, Subfamily B (ABCB/MDR/TAP), in the Japanese Population
4600/38J Hum Genet (2002) 47:38–50 N. Matsuda et al.: © Jpn EGF Soc receptor Hum Genet and osteoblastic and Springer-Verlag differentiation 2002 ORIGINAL ARTICLE Susumu Saito · Aritoshi Iida · Akihiro Sekine Yukie Miura · Chie Ogawa · Saori Kawauchi Shoko Higuchi · Yusuke Nakamura Three hundred twenty-six genetic variations in genes encoding nine members of ATP-binding cassette, subfamily B (ABCB/MDR/TAP), in the Japanese population Received: September 18, 2001 / Accepted: November 2, 2001 Abstract We screened DNAs from 48 Japanese individuals domain (Hyde et al. 1990). ABC proteins constitute a super- for single-nucleotide polymorphisms (SNPs) in nine genes family consisting of eight subfamilies: ABC1, MDR/TAP, encoding components of ATP-binding cassette subfamily CFTR/MRP, ALD, OABP, GCN20, WHITE, and ANSA B (ABCB/MDR/TAP) by directly sequencing the entire (Kerb et al. 2001; Human ABC gene nomenclature applicable genomic regions except for repetitive elements. committee, http://www.gene.ucl.ac.uk/nomenclature/ This approach identified 297 SNPs and 29 insertion/deletion genefamily/abc.html). polymorphisms among the nine genes. Of the 297 SNPs, 50 Members of the MDR/TAP subfamily include the were identified in the ABCB1 gene, 14 in TAP1, 35 in ATP-binding cassette, subfamily B (ABCB) and the TAP2, 48 in ABCB4, 13 in ABCB7, 21 in ABCB8, 21 in transporter associated with antigen processing (TAP). The ABCB9, 13 in ABCB10, and 82 in ABCB11. Thirteen were ABCB1 [ATP-binding cassette, subfamily B, member 1, located in 5Ј flanking regions, 237 in introns, 37 in exons, also called multidrug resistance (MDR)-1] gene encodes P- and 10 in 3Ј flanking regions. -
Transcriptional and Post-Transcriptional Regulation of ATP-Binding Cassette Transporter Expression
Transcriptional and Post-transcriptional Regulation of ATP-binding Cassette Transporter Expression by Aparna Chhibber DISSERTATION Submitted in partial satisfaction of the requirements for the degree of DOCTOR OF PHILOSOPHY in Pharmaceutical Sciences and Pbarmacogenomies in the Copyright 2014 by Aparna Chhibber ii Acknowledgements First and foremost, I would like to thank my advisor, Dr. Deanna Kroetz. More than just a research advisor, Deanna has clearly made it a priority to guide her students to become better scientists, and I am grateful for the countless hours she has spent editing papers, developing presentations, discussing research, and so much more. I would not have made it this far without her support and guidance. My thesis committee has provided valuable advice through the years. Dr. Nadav Ahituv in particular has been a source of support from my first year in the graduate program as my academic advisor, qualifying exam committee chair, and finally thesis committee member. Dr. Kathy Giacomini graciously stepped in as a member of my thesis committee in my 3rd year, and Dr. Steven Brenner provided valuable input as thesis committee member in my 2nd year. My labmates over the past five years have been incredible colleagues and friends. Dr. Svetlana Markova first welcomed me into the lab and taught me numerous laboratory techniques, and has always been willing to act as a sounding board. Michael Martin has been my partner-in-crime in the lab from the beginning, and has made my days in lab fly by. Dr. Yingmei Lui has made the lab run smoothly, and has always been willing to jump in to help me at a moment’s notice. -
Mitochondrial ABC Proteins in Health and Disease
View metadata, citation and similar papers at core.ac.uk brought to you by CORE provided by Elsevier - Publisher Connector Biochimica et Biophysica Acta 1787 (2009) 681–690 Contents lists available at ScienceDirect Biochimica et Biophysica Acta journal homepage: www.elsevier.com/locate/bbabio Mitochondrial ABC proteins in health and disease Ariane Zutz a, Simone Gompf a, Hermann Schägger b, Robert Tampé a,⁎ a Institute of Biochemistry, Biocenter, Goethe-University, Max-von-Laue-Str. 9, D-60348 Frankfurt a.M., Germany b Gustav-Embden-Zentrum of Biological Chemistry, Medical School, Goethe-University, Theodor-Stern-Kai 7, D-60590 Frankfurt a.M., Germany article info abstract Article history: ABC transporters represent one of the largest families of membrane proteins that are found in all three phyla Received 23 December 2008 of life. Mitochondria comprise up to four ABC systems, ABCB7/ATM1, ABCB10/MDL1, ABCB8 and ABCB6. Received in revised form 12 February 2009 These half-transporters, which assemble into homodimeric complexes, are involved in a number of key Accepted 13 February 2009 cellular processes, e.g. biogenesis of cytosolic iron–sulfur clusters, heme biosynthesis, iron homeostasis, Available online 24 February 2009 multidrug resistance, and protection against oxidative stress. Here, we summarize recent advances and emerging themes in our understanding of how these ABC systems in the inner and outer mitochondrial Keywords: fi Heme biosynthesis membrane ful ll their functions in important (patho) physiological processes, including -
The Role of Mitochondrial ATP-Binding Cassette Transporter
The Role of Mitochondrial ATP-Binding Cassette Transporter ABCB6 in Metabolism and Energy Balance By © 2019 Robert T Tessman Submitted to the graduate degree program in Toxicology and the Graduate Faculty of the University of Kansas in partial fulfillment of the requirements for the degree of Doctor of Philosophy. Committee Chair: Partha Kasturi, PhD Bruno Hagenbuch, PhD Hao Zhu, PhD Tiangang Li, PhD Luciano DiTacchio, PhD Date Defended: April 19th, 2019Title Page ii The dissertation committee for Robert T Tessman certifies that this is the approved version of the following dissertation: The role of Mitochondrial ATP-Binding Cassette Transporter ABCB6 in Metabolism and Energy Balance Committee Chair: Partha Kasturi, PhD Acceptance Page Date Approved: April 19th, 2019 iii Abstract Obesity and the associated health risks represent a world-wide health and financial crisis. Lack of physical activity combined with excessive caloric intake are the root cause of the problem. Despite the increased advocation for healthy lifestyle choices, the trend has yet to reverse and indeed, seems to be on the rise especially among pre- teens and adolescents, a constituent that had not been previously part of the obesity epidemic. Mitochondria are the “fuel-burners” of the body and like other combustion devices, become inefficient in the context of fuel surplus. Moreover, with chronic over-feeding, the physiological mechanisms that regulate energy balance become permanently dysfunctional leading to the progression of pathologies such as Type II diabetes and cardiovascular disease. Medical and scientific evidence confirms that mitochondria are integral to the responses necessary to adapt to over-nutrition. However, success in mitochondria- based therapies has been extremely limited in the context of metabolic diseases. -
Anti-ABCB8 (Aa 394-693) Polyclonal Antibody (DPABH-14194) This Product Is for Research Use Only and Is Not Intended for Diagnostic Use
Anti-ABCB8 (aa 394-693) polyclonal antibody (DPABH-14194) This product is for research use only and is not intended for diagnostic use. PRODUCT INFORMATION Antigen Description ABCB8 (ATP-binding cassette, sub-family B (MDR/TAP), member 8) is a protein-coding gene. Diseases associated with ABCB8 include acute myeloid leukemia, and myeloid leukemia, and among its related super-pathways are ABC-family proteins mediated transport and SLC- mediated transmembrane transport. GO annotations related to this gene include ATPase activity, coupled to transmembrane movement of substances and transporter activity. An important paralog of this gene is TAP2. Immunogen Recombinant fragment within Human ABCB8 aa 394-693. The exact sequence is proprietary. Genbank No.: BC141836.Sequence: LMSFLVA SQTVQRSMAN LSVLFGQVVR GLSAGARVFE YMALNPCIPL SGGCCVPKEQ LRGSVTFQNV CFSYPCRPGF EVLKDFTLTL PPGKIVALVG QSGGGKTTVA SLLERFYDPT AGVVMLD Isotype IgG Source/Host Rabbit Species Reactivity Mouse, Rat, Human Purification Immunogen affinity purified Conjugate Unconjugated Applications IHC-P, WB Format Liquid Size 100 μl Buffer pH: 7.30; Constituents: 50% Glycerol, 49% PBS, PBS (without Mg2+and Ca2+) Preservative 0.05% Sodium Azide Storage Shipped at 4°C. Store at 4°C short term (1-2 weeks). Upon delivery aliquot. Store at -20°C long term. Avoid freeze / thaw cycle. GENE INFORMATION 45-1 Ramsey Road, Shirley, NY 11967, USA Email: [email protected] Tel: 1-631-624-4882 Fax: 1-631-938-8221 1 © Creative Diagnostics All Rights Reserved Gene Name ABCB8 ATP-binding cassette,