Effects of Leachate Recirculation and Ph Adjustment
Total Page:16
File Type:pdf, Size:1020Kb
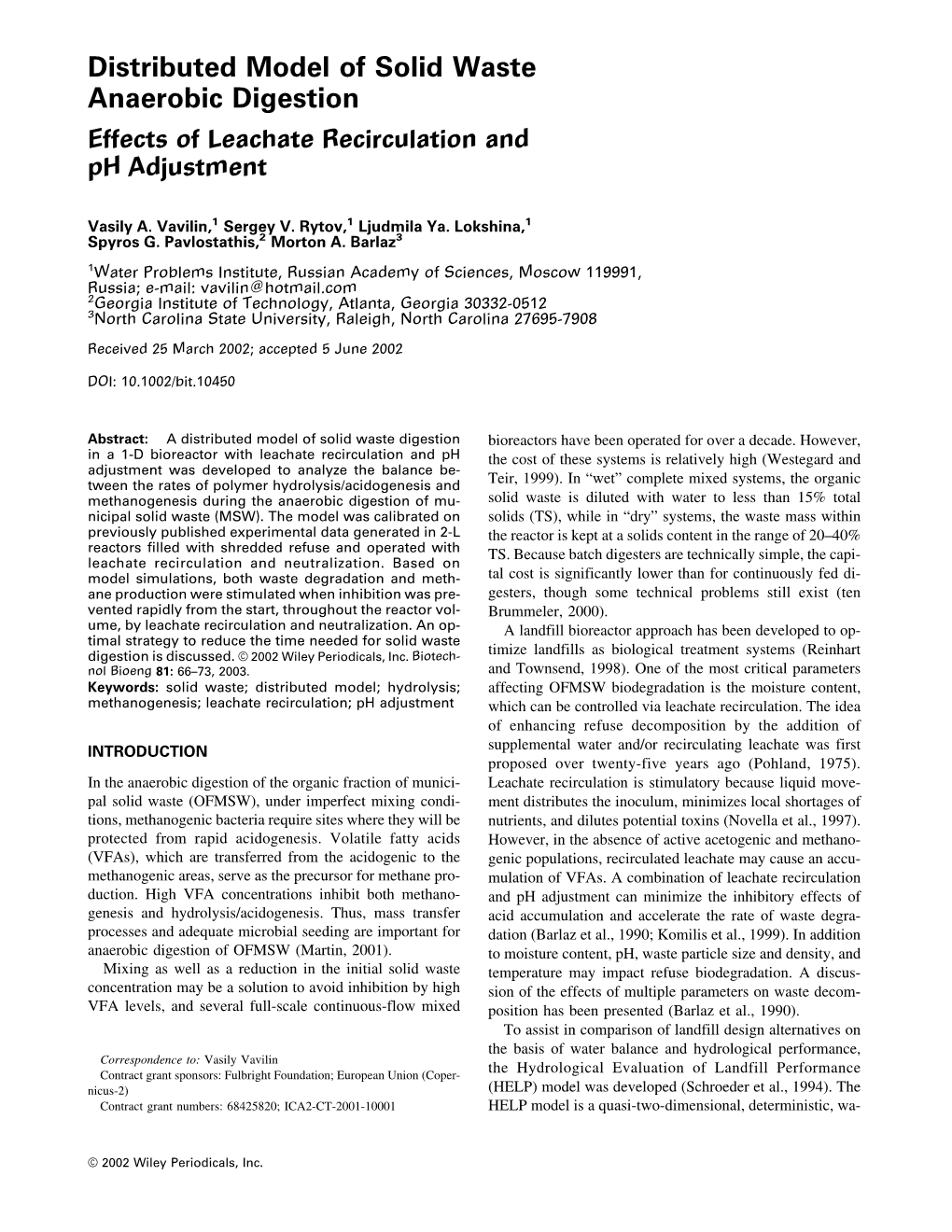
Load more
Recommended publications
-
Utilization of Ultraviolet-Visible Spectroscopy and Rheology for Sludge Characterization and Monitoring
Utilization of ultraviolet-visible spectroscopy and rheology for sludge characterization and monitoring by Jordan Smyth A thesis submitted to the Faculty of Graduate and Postdoctoral Affairs in partial fulfillment of the requirements for the degree of Master of Applied Science in Environmental Engineering Department of Civil and Environmental Engineering Ottawa-Carleton Institute for Environmental Engineering Carleton University Ottawa, Ontario © Jordan Smyth, 2018 Abstract Operation of sludge treatment processes mainly relies on manual control, which is far from ideal. There is a need for new approaches to optimize the operation of sludge treatment processes and wastewater plants. This research aims to identify new tools and methods that can be used for in- line and real-time characterization and monitoring of sludge. Two methods that were examined in this thesis that have potential to be used as monitoring technologies were ultraviolet/visible spectrophotometry and torque rheology. Effluent and filtrate absorbance measurements in the ultraviolet/visible range were successful in monitoring the progress of aerobic digestion. Torque rheology was not found to be sensitive enough for monitoring aerobic digestion of sludge, however it was able to detect changes in the total solids content of anaerobically digested sludge. Torque rheology detected significant changes in anaerobically digested sludge when trivalent cations were added, but not when divalent cations were added. i Acknowledgements First off, I would like to thank my supervisor Dr. Banu Ormeci. Her guidance, knowledge and motivation throughout the entire process was so helpful. She has had such a positive influence on my life and I will forever be grateful. I would also like to thank Dr. -
Anaerobic Treatment of Food Waste Leachate for Biogas Production Using a Novel Digestion System
Environ. Eng. Res. 2012 March,17(1) : 41-46 Research Paper http://dx.doi.org/10.4491/eer.2012.17.1.041 pISSN 1226-1025 eISSN 2005-968X Anaerobic Treatment of Food Waste Leachate for Biogas Production Using a Novel Digestion System Bong Su Lim1†, Byungchul Kim2, In Chung1 1Environmental Engineering, Daejeon University, Daejeon 300-716, Korea 2EnTechs, Seoul 135-240, Korea Abstract In this study, the performance of new digestion system (NDS) for the treatment of food waste leachate was evaluated. The food waste leachate was fed intermittently to an anaerobic reactor at increasing steps of 3.3 L/day (hydraulic retention time [HRT] = 30 day), 5 L/ day (HRT = 20 day), and finally 10 L/day (HRT = 10 day). In the anaerobic reactor, the pH and alkalinity were maintained at 7.6 to 8.2 and 8,940-14,400 mg/L, respectively. Maximum methane yield determined to be 0.686L CH4/g volatile solids (VS) containing HRT over 20 day. In the digester, 102,328 mg chemical oxygen demand (COD)/L was removed to produce 350 L/day (70% of the total) of biogas, but in the digested sludge reduction (DSR) unit, only 3,471 mg COD/L was removed with a biogas production of 158 L/day. Without adding any chemicals, 25% of total nitrogen (TN) and 31% of total phosphorus (TP) were removed after the DSR, while only 48% of TN and 32% of TP were removed in the nitrogen, phosphorus, and heavy metals (NPHM) removal unit. Total removal of TN was 73% and total removal of TP was 63%. -
More Than You Ever Wanted to Know About Your Aeration Septic System
More than you wanted to know about your Oldham brand Aeration Sewage Treatment System! Oldham brand aeration sewage treatment systems have an initial break-in period of six-to-eight weeks, during which time bacteria establish themselves in the unit. The development of these biological colonies occur naturally with the addition of sanitary wastes, so we recommend you use all your plumbing facilities in a normal manner from initial start-up. You may notice a septic odor during this initial break in period, this should clear up within 2-3 weeks. You may also notice a tendency for the unit to foam from laundry wastes during this period. This is normal and it should cease by the sixth week. You can help by using only moderate amounts of low-sudsing biodegradable detergents. An Oldham brand aeration sewage treatment system, operating properly and well maintained will NOT smell bad. The smell should be similar to what it smells like after a farmer plows their field, a kind of musty-earthy smell. The color in the aeration chamber should be chocolate brown. If a system smells bad, it is probably not operating long enough (or not at all). See below for how long it should run. Sewage treatment is a multi-stage process to renovate wastewater before it reenters a body of water. The goal is to reduce or remove organic matter, solids, nutrients, disease-causing organisms and other pollutants from wastewater. Suggested Detergents, Bleaches & Toilet Paper for use in an Oldham Aeration Septic System Detergents (for non HE machines) Bleaches Cleaning products: Recommend using non- All HE detergents are acceptable (only use powdered) chlorine, non-ammonia, non-antibacterial, non- Should be concentrated, powdered, low- Biz toxic and bio-degradable. -
Anaerobic / Aerobic Digestion for Enhanced Solids and Nitrogen Removal
Anaerobic / Aerobic Digestion for Enhanced Solids and Nitrogen Removal Sarita Banjade Thesis submitted to the faculty of the Virginia Polytechnic Institute and State University in partial fulfillment of the requirements for the degree of Master of Science In Environmental Engineering Dr. John T. Novak, Chair Dr. Gregory D. Boardman Dr. Clifford W. Randall December 4, 2008 Blacksburg, VA Keywords: mesophilic anaerobic digestion, aerobic digestion, solids removal, nitrogen removal, dewatering and biosolids odors Copyright © 2008, Sarita Banjade Anaerobic / Aerobic Digestion for Enhanced Solids and Nitrogen Removal Sarita Banjade Abstract Anaerobic digestion of wastewater sludge has widely been in application for stabilization of sludge. With the increase in hauling cost and many environmental and health concerns regarding land application of biosolids, digestion processes generating minimized sludge with better effluent characteristics is becoming important for many public and wastewater utilities. This study was designed to investigate the performance of anaerobic-aerobic-anaerobic digestion of sludge and compare it to anaerobic-aerobic digestion and single stage mesophilic digestion of sludge. Experiments were carried out in three stages: Single-stage mesophilic anaerobic digestion (MAD) 20d SRT; Sequential Anaerobic/Aerobic digestion (Ana/Aer); and Anaerobic/Aerobic/Anaerobic digestion (An/Aer/An). The Anaerobic/Aerobic/Anaerobic digestion of sludge was studied with two options to determine the best option in terms of effluent characteristics. The two sludge withdrawal options were to withdraw effluent from the anaerobic digester (An/Aer/An –A) or withdraw effluent from the aerobic digester (An/Aer/An – B). Different operational parameters, such as COD removal, VS destruction, biogas production, Nitrogen removal, odor removal and dewatering properties of the resulting biosolids were studied and the results were compared among different processes. -
THE EFFECTS of Ph on AEROBIC SLUDGE DIGESTION by Herbert
THE EFFECTS OF pH ON AEROBIC SLUDGE DIGESTION by Herbert Randolph Moore Thesis submitted to the Graduate Faculty of the Virginia Polytechnic Insti'tute and State University in partial fulfillment of the requirements for the degree of MASTER OF SCIENCE in Sanitary Engineering APPROVED: Dr. c~ w. Randall, Chairman Pl)()W(IJ. J. Cibul ka Dr. R. E; Benoit September 1970 Blacksburg, Virginia ACKNOWLEDGMENTS The author wishes to express his appreciation for the constant . guidance and constructive criticism offered by his thesis· advisor, Dr. Cl ifford W. Randall, throughout the preparation of this text •. He would also like to express his appreciation to.Dr. Ernest M. Jennelle, Dr. Paul H. King, Professor H. Grady Callison ~nd Professor -J•. J. · Cibul ka for their encouragement and counsel throughout" the grad- · . uate program, to and Dr. Ernest M. Jennelle,.for their valuable assistance in the laboratory, and to for the typing and proofreadfog of this. text. ~- To his wife, he wishes to ·express his fondest appreciation and. grat- itude for the understanding, f~ith and patience she has shown and for providing the home atmosphere that has made it all worth the effort • . This research was supported by a fellowship from the United States . Public Health Service. TABLE OF CONTENTS Page I. ACKNOWLEDGMENTS (I Cl • fl Q ti Q • • • • • ii IL LIST OF TABLES • . • • v III. LIST OF FIGURES I • vi IV. ·INTRODUCTION ••••• l A•. Biological Aspects of Aerobic Sludge Digestion • . l B. Purpose of Research Investigation . 3 V. LITERATURE REVIEW ••• . 5 VI.· METHODS AND MATERIALS o fl e e • o Q • 14 A. -
Treating Industrial Wastewater : Anaerobic Digestion Comes Of
Cover Story TREATING INDUSTRIAL WASTEWATER: Anaerobic DigeSlio Aerobic Anaerobic ---' Effluent, Effluent, C02, ) -"\.4% 10% Comes of Age ~48% / , ~ . ~ i 4JI'// ~ Anaer bic tr atment systems offer import nl ~ / : . advant ges over conventionally applied .:' . -1.1 m.llkg-COD +4.3 M.llkg-COD aerobic processes for removing org nic +31 g NH4-N/kg-COD +3.2 g NH4-N/kg-COD pollutant from aler-based stre ms FIGURE 1. This comparison shows the respective fate of organic materials that are biodegraded under aerobic versus anaerobic conditions. Aerobic treat Robbert Kleerebezem , Technical University of Delft ment requires energy input for aeration, whereas a and Herve Macarie, Institut de Recherche pour le Developpement net energy surplus is generated during anaerobic treatment, in the form of methane-bearing biogas that can be used to power utility boilers onsite n the absence of molecular oxygen, wastewater constituents on anaerobic the production of byproduct methane natural environments depend on microbes. As a result, it is the opinion rich biogas. The use of the methane for the activity of anaerobic microor of these authors that such systems are energy generation elsewhere at the ganisms for the biological degrada not currently being applied as widely plant allows for conservation of more tion of organic substrates. In as they could be within the global than 90% of the caloric value of the or Ianaerobic environments where, other chemical process industries (CPl). ganic substrates being treated. In addi than carbon dioxide, no inorganic elec This article aims to address some of tion, modern anaerobic bioreactors do tron acceptors are present, the final the lingering misconceptions associ not require large energy input for me degradation of organic compounds is ated with anaerobic wastewater treat chanical mixing (which is required to achieved by their conversion to ment. -
Aerobic Digestion, Or, It’S a Bug Eat Bug World
Aerobic Digestion, or, It’s a Bug eat Bug World Brett Ward Municipal Technical Advisory Service The University of Tennessee Aerobic Digestion • Continuation of the Activated Sludge Process – “Super” Extended Aeration – No food is added – Reduction of Volatile Solids through: • Endogenous Respiration – Bacterial Cells use their own protoplasm for energy – Some cells die and become food for others Biological Chemistry • BOD, Bug Food, Organic Pollution – Test of the organic strength of sewage • Organic- from plants and animals – Protein, carbohydrates, fats • Fecal matter, food scrapes, paper, industrial waste of plant or animal origin. • Normal Bacteriological Respiration BOD + BOD Bugs + O2 = More BOD Bugs + CO2 + H20 + NH3 Respiration / Oxidation • Respiration – We call it breathing – Scientifically it is the release of energy – The Bugs want the energy in the BOD • Energy to live and grow – The Bugs are teeny tiny chemical factories • They split complex organic compounds into simple oxidized compounds. Respiration / Oxidation • Normal Bacteriological Respiration BOD + BOD Bugs + O2 = More BOD Bugs + CO2 + H20 + NH3 Glucose C6H12O6+ BOD Bugs+ O2 = More BOD Bugs+ CO2+ H20 Protein C3H7O2N + BODBugs+ O2= More BODBugs+ CO2+ H20 + NH3 Sewage Treatment • Sewage, BOD – Carbs, Protein, Fats • Add air and mix well • Bugs grow – And Grow •And Grow Waste excess Bugs to Digester • With out wasting MLSS goes up, up, up and OUT! • Waste today what grew today Biological Chemistry • Digesters receive no food or BOD • Endogenous Respiration BOD Bugs +O2 = Less BOD Bugs +CO2 + H2O + NH3 • Bugs use stored energy • Some die, and become food for others • Reduction of Volatile Solids Digestion Goals Thicken Waste Activated Sludge: Decanting Control Odors, Keep it aerobic Reduce Sludge Volume, Endogenous Resp. -
AEROBIC DIGESTION Not the Same Old Same Old
AEROBIC DIGESTION Not the Same Old Same Old Paul Bizier, PE Barge Design Solutions, Inc. – Nashville, Tennessee Aerobic Digestion Not Treatment of Raw Waste Endogenous Respiration All Bugs + No Food = Cannibalism Reduces Volatile Solids Kills Pathogenic Organisms Advantages Disadvantages Lower capital costs for facilities with Higher operating costs and other capacities less than 19,000 m3/day operational issues when treating Aerobic Digestion (5 MGD) primary sludge Minimal nuisance odors (except for Higher energy costs than other short SRT ATAD processes) stabilization processes, especially traditional mesophilic anaerobic Pros and Cons digestion Simple construction Limited pathogen reduction (except for ATAD) No danger of explosions or Lower cake solids (except for some suffocations ATAD processes) Simple operation Potential for alkalinity depletion if nitrification occurs Weaker sidestreams Less impact from low pH What is the End Use? Vector Attraction Reduction Criteria Public Access? Marketing/Distribution? • • Class A Pathogen Class A Pathogen Reduction Reduction • EQ Class Requirements Restricted Access? • Class B Pathogen Reduction Landfill? Aerobic Digestion and Pathogen Reduction • Class A Pathogen Reduction • PFRP Processes • Thermophilic Aerobic Digestion • PFRP-Equivalent Processes • ATAD – Autothermal Thermophilic Aerobic Digestion • Alternative 1 – Time/Temperature (Thermophilic Systems) • Class B Pathogen Reduction • PSRP - Specifically Defined Operating Criteria (40 CFR 257) • Aerobic Digestion • Alternative 1 - Testing What’s Not Listed? Unless Defined Criteria Are Met, Alternative Processes – NOT LISTED Digestion Processes Are Not PFRP or Thickened Aerobic Digestion PSRP Modifications For PFRP Aerobic/Anoxic Digestion May Meet Alternative 1, if Process is Thermophilic Aerobic/Anaerobic Digestion Most Proprietary Processes May Meet Alternative 4 (Testing) Less Desirable Autothermal thermophilic aerobic digestion (ATAD) is listed as a PFRP process. -
Aerobic Digesters Anaerobic Digesters Aerobic Digesters
6/8/2012 Aerobic Digesters Anaerobic Digesters Doug Hill www.dhillenvironmental.com Purpose of Digestion Stabilize Solids Minimize Odors Volume Reduction Improve Dewaterability Meet Part 503 Land Application Requirements Vector Attraction Reduction Pathogens Reduction Aerobic Digesters 1 6/8/2012 Lag Log Declining Endogenous Growth Growth Growth Growth Food Conventional Treatment Extended Air Growth Rate ofOrganisms Rate Growth Sludge Production Cell Residence Time Oxygen Respiration CO 2 Energy Cell BOD Maintenance Heat, Cell Growth New Biomass Bacterial Cell Aerobic Metabolism Oxygen Respiration CO 2 Energy Stored Cell BOD Food Maintenance Cell Components Heat, Cell Growth New Biomass Bacterial Cell Endogenous Respiration – Survival Mode Stored Food Used Cell Growth Rate Cell Components Used Reduced 2 6/8/2012 Endogenous Respiration Oxygen – Cell Death Respiration CO 2 Energy Stored Cell Food Maintenance Cell Components Bacterial Cell Stored Food Used Cell Components Used Cell Dies and Splits Open (Aerobic Digestion) Aerobic Digestion Endogenous Stabilization High CRT allows for the microbes to feed off of the cell contents of other dying/decaying microbes under digestion. Not all solids can be digested 20 to 25% by weight inert solids Fine inorganic solids, organic solids, and cell components that are not degradable Aerobic Digestion ADVANTAGES The process is easy to control, easy start-up Low ammonia and BOD in return stream Few odors are experienced if properly designed and operated Explosive gases (methane) are not produced 3 6/8/2012 Aerobic Digestion DISADVANTAGES Aerobic digestion does NOT produce energy Aerobic digestion process is energy intensive Not usually used for primary sludge due to high O 2 demand and additional biomass produced Temperature variability throughout the year causes variability in the operating performance Stabilized sludge may be difficult to dewater Aerobic Digestion OPERATION Batch Operation: Add sludge Digest – mix, aerate Turn off the aeration system Allow the solids to settle Decant the clear liquid Control: D.O. -
Lesson B5 SEWAGE SLUDGE TREATMENT
EMWATER E-LEARNING COURSE PROJECT FUNDED BY THE EUROPEAN UNION LESSON B5: SEWAGE SLUDGE TREATMENT Lesson B5 SEWAGE SLUDGE TREATMENT Authors: Holger Pabsch Claudia Wendland Institute of Wastewater Management Hamburg University of Technology Keywords sewage sludge, stabilisation, sludge thickening, sludge dewatering, sludge pumping, digestion Page 1 of 23 EMWATER E-LEARNING COURSE PROJECT FUNDED BY THE EUROPEAN UNION LESSON B5: SEWAGE SLUDGE TREATMENT Table of Content Overview and summary of this Lesson 3 1. Sewage sludge quantity and characteristics 4 2. Sludge pumping systems 5 3. Sludge stabilisation 7 3.1 Simultaneous aerobic stabilisation 7 3.2 Mesophilic anaerobic digestion 8 3.3 Aerobic digestion 11 3.4 Alkaline Stabilisation 12 4. Thickening/ dewatering of Sludge 14 4.1 Dimensioning of a static thickener 15 4.2 Dewatering 16 5. Sludge Disposal and Agricultural Utilisation 20 6. Exercises 21 7. References and further Information 23 Page 2 of 23 EMWATER E-LEARNING COURSE PROJECT FUNDED BY THE EUROPEAN UNION LESSON B5: SEWAGE SLUDGE TREATMENT Overview and summary of this Lesson Sludge originates from the process of treatment of wastewater and is separated from the treatment process by sedimentation or flotation. Sewage sludge consists of water and solids that can be divided into mineral and organic solids. The quantity and characteristics of sludge depend very much on the treatment processes. Most of the pollutants that enter the wastewater get adsorbed to the sewage sludge. Therefore, sewage sludge contains pathogens (and heavy metals, many organic pollutants pesticides, hydrocarbons etc. if the sewage contains industrial influence). Sludge is, however, rich in nutrients such as nitrogen and phosphorous and contains valuable organic matter that is useful if soils are depleted or subject to erosion. -
Autothermal Thermophilic Aerobic Digestion (ATAD) for Heat, Gas, and Production of a Class a Biosolids with Fertilizer Potential
microorganisms Review Autothermal Thermophilic Aerobic Digestion (ATAD) for Heat, Gas, and Production of a Class A Biosolids with Fertilizer Potential J. Tony Pembroke and Michael P. Ryan * Department of Chemical Sciences, School of Natural Sciences and Bernal Institute, University of Limerick, V94 T9PX Limerick, Ireland * Correspondence: [email protected]; Tel.: +353-61-234730 Received: 28 June 2019; Accepted: 23 July 2019; Published: 25 July 2019 Abstract: Autothermal thermophilic aerobic digestion (ATAD) is a microbial fermentation process characterized as a tertiary treatment of waste material carried out in jacketed reactors. The process can be carried out on a variety of waste sludge ranging from human, animal, food, or pharmaceutical waste where the addition of air initiates aerobic digestion of the secondary treated sludge material. Digestion of the sludge substrates generates heat, which is retained within the reactor resulting in elevation of the reactor temperature to 70–75 ◦C. During the process, deamination of proteinaceous materials also occurs resulting in liberation of ammonia and elevation of pH to typically pH 8.4. These conditions result in a unique microbial consortium, which undergoes considerable dynamic change during the heat-up and holding phases. The change in pH and substrate as digestion occurs also contributes to this dynamic change. Because the large reactors are not optimized for aeration, and because low oxygen solubility at elevated temperatures occurs, there are considerable numbers of anaerobes recovered which also contributes to the overall digestion. As the reactors are operated in a semi-continuous mode, the reactors are rarely washed, resulting in considerable biofilm formation. Equally, because of the fibrous nature of the sludge, fiber adhering organisms are frequently found which play a major role in the overall digestion process. -
Solids Handling and Disposal
Wastewater Treatment Plant Operator Certification Training Module 6: Solids Handling and Disposal This course includes content developed by the Pennsylvania Department of Environmental Protection (Pa. DEP) in cooperation with the following contractors, subcontractors, or grantees: The Pennsylvania State Association of Township Supervisors (PSATS) Gannett Fleming, Inc. Dering Consulting Group Penn State Harrisburg Environmental Training Center MODULE 6: SOLIDS HANDLING AND DISPOSAL Topical Outline Unit 1 – Sludge Thickening I. Purpose of Sludge Thickening II. Gravity Thickeners III. Dissolved Air Flotation Thickeners IV. Centrifuge Thickeners V. Gravity Belt Thickeners Unit 2 – Anaerobic Digestion I. Purpose of Digestion A. General Overview B. How Digestion Works II. Components of an Anaerobic Digester System A. Anaerobic Digester B. Gas System III. Operations Overview of Anaerobic Digestion A. Starting and Feeding a Digester B. Mixing Tank Contents C. Gas Production D. Removing Tank Contents IV. Strategies for Maintaining an Anaerobic Digestion System A. Test Interpretation and Controls B. Checklists C. Troubleshooting D. Digester Cleaning E. Cleaning Pipelines and Valves Bureau of Water Supply and Wastewater Management, Department of Environmental Protection i Wastewater Treatment Plant Operator Training MODULE 6: SOLIDS HANDLING AND DISPOSAL Unit 3 – Aerobic Digestion I. Aerobic Digestion A. Overview B. Comparisons between Anaerobic and Aerobic Digestion C. Process Description D. Operation E. Records F. Problems Unit 4 – Solids Management Planning I. Digested Sludge Handling A. Overview B. Drying Beds C. Reed Beds D. Lagoon E. Mechanical Dewatering II. Sludge Disposal A. Methods of Sludge Disposal B. Regulations III. Review of Plans and Specifications A. Items to Review Bureau of Water Supply and Wastewater Management, Department of Environmental Protection ii Wastewater Treatment Plant Operator Training Unit 1 – Sludge Thickening Learning Objectives Describe the purpose of sludge thickening.