Flannel Flower)
Total Page:16
File Type:pdf, Size:1020Kb
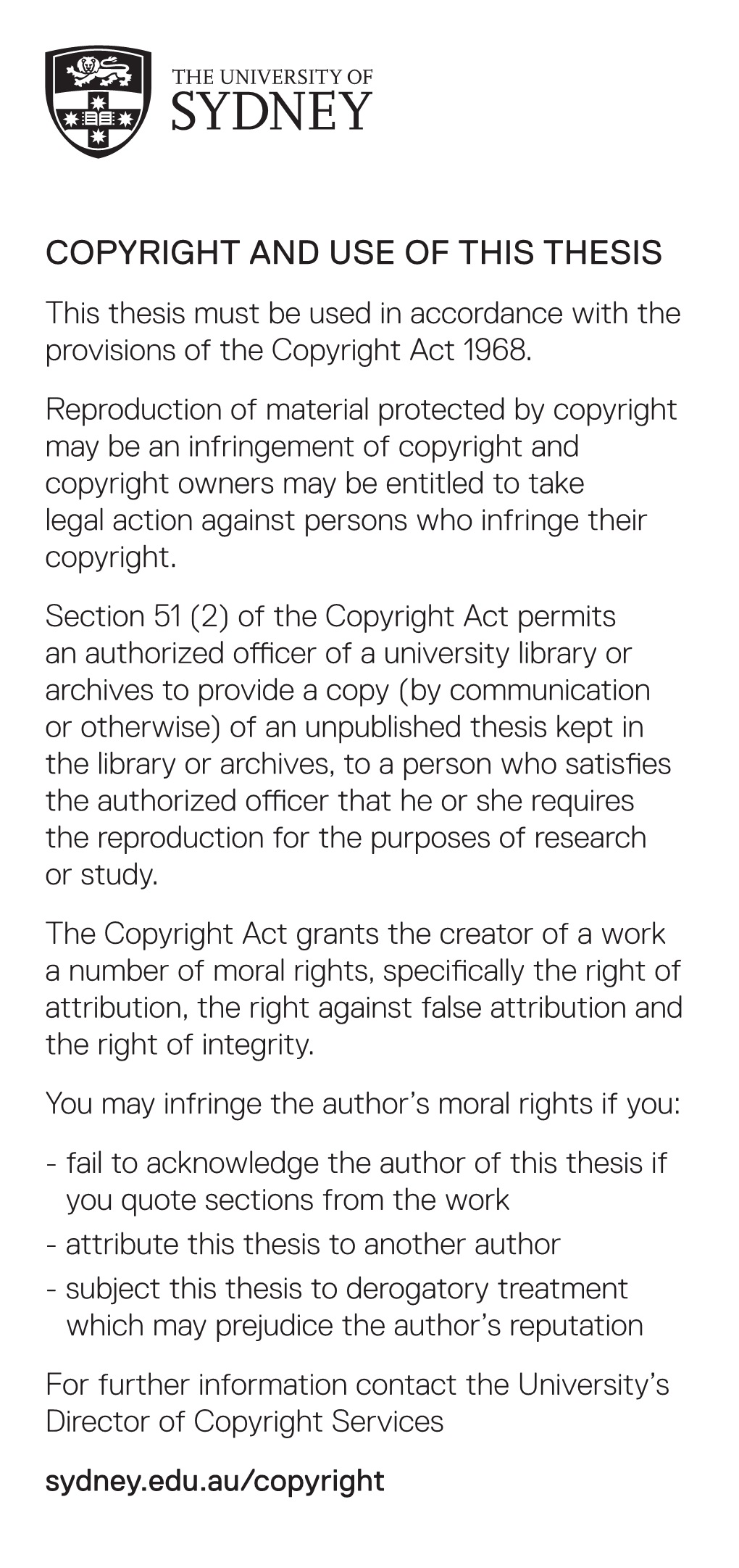
Load more
Recommended publications
-
Newsletter No.67
ISSN 0818 - 335X November, 2003 ASSOCIATION OF SOCIETIES FOR GROWING AUSTRALIAN PLANTS ABN 56 654 053 676 THE AUSTRALIAN DAISY STUDY GROUP NEWSLETTER NO. 67 Esma Salkin Studentship and proposed projects for the studentship Leader's letter and coming events Species or forms new to members Jeanette Closs, Ozotharnnus reflexifolius Judy Barker and Joy Greig Daisies of Croajingolong N. P. (contd.) Joy Greig More about Xerochrysum bracteaturn Barrie Hadlow from Sandy Beach (NSW) A postscript to 'Daisies in the Vineyard' Ros Cornish Leptorhynchos sprfrom-Dimmocks -Judy Barker Lookout Daisies on Lord Howe Island Pat and John Webb Ozothamnus rodwayi Beryl Birch Daisies for the SA Plant Sale on ~7~~128'~Syd and Syl Oats September Report from Pomonal Linda Handscombe ADSG Display at the APS SA Plant Sale Syd and Syl Oats Propagation pages - Ray Purches, Bev Courtney, Margaret Guenzel, Syd Oats, Judy Barker An innovative use for a rabbit's cage Syd and Syd Oats Members' reports - Corinne Hampel, Jeff Irons, Ray Purches, Jan Hall, Ros Cornish, Jeanette Closs, Syd Oats, Gloria Thomlinson June Rogers Podolepis robusta Financial Report, editor's letter, new (illustrated by Gloria Thomlinson) members, seed donors, seed additions and deletions, index for 2003 newsletters OFFICE BEARERS: Leader and ADSG Herbarium Curator -Joy Greig, PO Box 258, Mallacoota, 3892. TellFax: (03) 51 58 0669 (or Unit 1, 1a Buchanan St, Boronia, 31 55. Tel: (03) 9762 7799) Email [email protected] Treasurer - Bev Courtney, 9 Nirvana Close, Langwarrin, 3910. Provenance Seed Co-ordinator - Maureen Schaumann, 88 Albany Drive, Mulgrave, 3170. Tel: (03) 9547 3670 Garden and Commercial Seed Co-ordinator and Interim Newsletter Editor: - Judy Barker, 9 Widford St, East Hawthorn, 3123. -
Computer-Assisted Recovery of Threatened Plants: Keys for Breaking Seed Dormancy of Eryngium Viviparum
ORIGINAL RESEARCH published: 12 December 2017 doi: 10.3389/fpls.2017.02092 Computer-Assisted Recovery of Threatened Plants: Keys for Breaking Seed Dormancy of Eryngium viviparum Manuel Ayuso 1, Pablo Ramil-Rego 2, Mariana Landin 3, Pedro P. Gallego 1* and M. Esther Barreal 1 1 Applied Plant and Soil Biology, Faculty of Biology, University of Vigo, Vigo, Spain, 2 GI-1934 TB-Biodiversity, IBADER, Universidad de Santiago, Lugo, Spain, 3 Department of Pharmacology, Pharmacy and Pharmaceutical Technology, Faculty of Pharmacy, University of Santiago de Compostela, Santiago de Compostela, Spain Many endangered plants such as Eryngium viviparum (Apiaceae) present a poor Edited by: Juan Caballero, germination rate. This fact could be due to intrinsic and extrinsic seed variability Universidad Autónoma de Querétaro, influencing germination and dormancy of seeds. The objective of this study is to Mexico better understand the physiological mechanism of seed latency and, through artificial Reviewed by: Emiliano Villordo Pineda, intelligence models, to determine the factors that stimulate germination rates of Polytechnic University of Guanajuato, E. viviparum seeds. This description could be essential to prevent the disappearance Mexico of endangered plants. Germination in vitro was carried out under different dormancy Renato Vicentini, Universidade Estadual de Campinas, breaking and incubation procedures. Percentages of germination, viability and E:S ratio Brazil were calculated and seeds were dissected at the end of each assay to describe embryo *Correspondence: development. The database obtained was modeled using neurofuzzy logic technology. Pedro P. Gallego [email protected] We have found that the most of Eryngium seeds (62.6%) were non-viable seeds (fully empty or without embryos). -
Apiaceae) - Beds, Old Cambs, Hunts, Northants and Peterborough
CHECKLIST OF UMBELLIFERS (APIACEAE) - BEDS, OLD CAMBS, HUNTS, NORTHANTS AND PETERBOROUGH Scientific name Common Name Beds old Cambs Hunts Northants and P'boro Aegopodium podagraria Ground-elder common common common common Aethusa cynapium Fool's Parsley common common common common Ammi majus Bullwort very rare rare very rare very rare Ammi visnaga Toothpick-plant very rare very rare Anethum graveolens Dill very rare rare very rare Angelica archangelica Garden Angelica very rare very rare Angelica sylvestris Wild Angelica common frequent frequent common Anthriscus caucalis Bur Chervil occasional frequent occasional occasional Anthriscus cerefolium Garden Chervil extinct extinct extinct very rare Anthriscus sylvestris Cow Parsley common common common common Apium graveolens Wild Celery rare occasional very rare native ssp. Apium inundatum Lesser Marshwort very rare or extinct very rare extinct very rare Apium nodiflorum Fool's Water-cress common common common common Astrantia major Astrantia extinct very rare Berula erecta Lesser Water-parsnip occasional frequent occasional occasional x Beruladium procurrens Fool's Water-cress x Lesser very rare Water-parsnip Bunium bulbocastanum Great Pignut occasional very rare Bupleurum rotundifolium Thorow-wax extinct extinct extinct extinct Bupleurum subovatum False Thorow-wax very rare very rare very rare Bupleurum tenuissimum Slender Hare's-ear very rare extinct very rare or extinct Carum carvi Caraway very rare very rare very rare extinct Chaerophyllum temulum Rough Chervil common common common common Cicuta virosa Cowbane extinct extinct Conium maculatum Hemlock common common common common Conopodium majus Pignut frequent occasional occasional frequent Coriandrum sativum Coriander rare occasional very rare very rare Daucus carota Wild Carrot common common common common Eryngium campestre Field Eryngo very rare, prob. -
Flowering Plants Eudicots Apiales, Gentianales (Except Rubiaceae)
Edited by K. Kubitzki Volume XV Flowering Plants Eudicots Apiales, Gentianales (except Rubiaceae) Joachim W. Kadereit · Volker Bittrich (Eds.) THE FAMILIES AND GENERA OF VASCULAR PLANTS Edited by K. Kubitzki For further volumes see list at the end of the book and: http://www.springer.com/series/1306 The Families and Genera of Vascular Plants Edited by K. Kubitzki Flowering Plants Á Eudicots XV Apiales, Gentianales (except Rubiaceae) Volume Editors: Joachim W. Kadereit • Volker Bittrich With 85 Figures Editors Joachim W. Kadereit Volker Bittrich Johannes Gutenberg Campinas Universita¨t Mainz Brazil Mainz Germany Series Editor Prof. Dr. Klaus Kubitzki Universita¨t Hamburg Biozentrum Klein-Flottbek und Botanischer Garten 22609 Hamburg Germany The Families and Genera of Vascular Plants ISBN 978-3-319-93604-8 ISBN 978-3-319-93605-5 (eBook) https://doi.org/10.1007/978-3-319-93605-5 Library of Congress Control Number: 2018961008 # Springer International Publishing AG, part of Springer Nature 2018 This work is subject to copyright. All rights are reserved by the Publisher, whether the whole or part of the material is concerned, specifically the rights of translation, reprinting, reuse of illustrations, recitation, broadcasting, reproduction on microfilms or in any other physical way, and transmission or information storage and retrieval, electronic adaptation, computer software, or by similar or dissimilar methodology now known or hereafter developed. The use of general descriptive names, registered names, trademarks, service marks, etc. in this publication does not imply, even in the absence of a specific statement, that such names are exempt from the relevant protective laws and regulations and therefore free for general use. -
Conserving Europe's Threatened Plants
Conserving Europe’s threatened plants Progress towards Target 8 of the Global Strategy for Plant Conservation Conserving Europe’s threatened plants Progress towards Target 8 of the Global Strategy for Plant Conservation By Suzanne Sharrock and Meirion Jones May 2009 Recommended citation: Sharrock, S. and Jones, M., 2009. Conserving Europe’s threatened plants: Progress towards Target 8 of the Global Strategy for Plant Conservation Botanic Gardens Conservation International, Richmond, UK ISBN 978-1-905164-30-1 Published by Botanic Gardens Conservation International Descanso House, 199 Kew Road, Richmond, Surrey, TW9 3BW, UK Design: John Morgan, [email protected] Acknowledgements The work of establishing a consolidated list of threatened Photo credits European plants was first initiated by Hugh Synge who developed the original database on which this report is based. All images are credited to BGCI with the exceptions of: We are most grateful to Hugh for providing this database to page 5, Nikos Krigas; page 8. Christophe Libert; page 10, BGCI and advising on further development of the list. The Pawel Kos; page 12 (upper), Nikos Krigas; page 14: James exacting task of inputting data from national Red Lists was Hitchmough; page 16 (lower), Jože Bavcon; page 17 (upper), carried out by Chris Cockel and without his dedicated work, the Nkos Krigas; page 20 (upper), Anca Sarbu; page 21, Nikos list would not have been completed. Thank you for your efforts Krigas; page 22 (upper) Simon Williams; page 22 (lower), RBG Chris. We are grateful to all the members of the European Kew; page 23 (upper), Jo Packet; page 23 (lower), Sandrine Botanic Gardens Consortium and other colleagues from Europe Godefroid; page 24 (upper) Jože Bavcon; page 24 (lower), Frank who provided essential advice, guidance and supplementary Scumacher; page 25 (upper) Michael Burkart; page 25, (lower) information on the species included in the database. -
Its Active Constituents, Pharmacological Uses, Traditional and Potential Benefits
Pharmacophore, 11(2) 2020, Pages: 40-52 Pharmacophore ISSN-2229-5402 Journal home page: http://www.pharmacophorejournal.com A DETAILED REVIEW ON THE RARELY FOUND HIMALAYAN HERB SELINUM VAGINATUM: ITS ACTIVE CONSTITUENTS, PHARMACOLOGICAL USES, TRADITIONAL AND POTENTIAL BENEFITS Nikita Saraswat1*, Neetu Sachan2, Phool Chandra3 1. Pharmacy Department, Pranveer Singh Institute of Technology, Kanpur-Agra-Delhi National Highway-2, Bhauti, Kanpur (UP)-209 305, India. 2. Department of Pharmaceutical Chemistry, School of Pharmaceutical Sciences, IFTM University, Lodhipur Rajput, Delhi Road, (NH-24), Moradabad (UP)-244 102, India. 3. Department of Physiology & Pharmacology School of Pharmaceutical Sciences, IFTM University, Lodhipur Rajput, Delhi Road, (NH-24), Moradabad (UP)-244 102, India. ARTICLE I N F O A B S T R A C T Received: Introduction: Traditional herbs and drugs are the current choices of people in most areas 29 Sep 2019 where herbs are commonly being prescribed and used for the management of diseases. The Received in revised form: current scenario of undertaking Ayurveda medications and herbs as a potent cure for many 22 Mar 2020 diseases is growing phenomenally. In this paper, we have presented a detailed analysis of a Himalayan Herb Selinum vaginatum, which is a good source and a potential treatment for Accepted: curing many diseases. The potential of this herb is not much explored scientifically. It is found 02 Apr 2020 in the regions of Northern Himalayas alongside the states like Himachal Pradesh, Kashmir, Available online: Uttarakhand, high altitude regions China mainland (Himalayan region) Kailash Mountain 28 Apr 2020 region, Pakistan, etc. Objectives: The main objective of the paper is to get detailed information about the taxonomical classification of species, traditional uses, pharmacological– actions, and chemical composition of Selinum vaginatum. -
Rare Or Threatened Vascular Plant Species of Wollemi National Park, Central Eastern New South Wales
Rare or threatened vascular plant species of Wollemi National Park, central eastern New South Wales. Stephen A.J. Bell Eastcoast Flora Survey PO Box 216 Kotara Fair, NSW 2289, AUSTRALIA Abstract: Wollemi National Park (c. 32o 20’– 33o 30’S, 150o– 151oE), approximately 100 km north-west of Sydney, conserves over 500 000 ha of the Triassic sandstone environments of the Central Coast and Tablelands of New South Wales, and occupies approximately 25% of the Sydney Basin biogeographical region. 94 taxa of conservation signiicance have been recorded and Wollemi is recognised as an important reservoir of rare and uncommon plant taxa, conserving more than 20% of all listed threatened species for the Central Coast, Central Tablelands and Central Western Slopes botanical divisions. For a land area occupying only 0.05% of these divisions, Wollemi is of paramount importance in regional conservation. Surveys within Wollemi National Park over the last decade have recorded several new populations of signiicant vascular plant species, including some sizeable range extensions. This paper summarises the current status of all rare or threatened taxa, describes habitat and associated species for many of these and proposes IUCN (2001) codes for all, as well as suggesting revisions to current conservation risk codes for some species. For Wollemi National Park 37 species are currently listed as Endangered (15 species) or Vulnerable (22 species) under the New South Wales Threatened Species Conservation Act 1995. An additional 50 species are currently listed as nationally rare under the Briggs and Leigh (1996) classiication, or have been suggested as such by various workers. Seven species are awaiting further taxonomic investigation, including Eucalyptus sp. -
Plant List for VC54, North Lincolnshire
Plant List for Vice-county 54, North Lincolnshire 3 Vc61 SE TA 2 Vc63 1 SE TA SK NORTH LINCOLNSHIRE TF 9 8 Vc54 Vc56 7 6 5 Vc53 4 3 SK TF 6 7 8 9 1 2 3 4 5 6 Paul Kirby, 31/01/2017 Plant list for Vice-county 54, North Lincolnshire CONTENTS Introduction Page 1 - 50 Main Table 51 - 64 Summary Tables Red Listed taxa recorded between 2000 & 2017 51 Table 2 Threatened: Critically Endangered & Endangered 52 Table 3 Threatened: Vulnerable 53 Table 4 Near Threatened Nationally Rare & Scarce taxa recorded between 2000 & 2017 54 Table 5 Rare 55 - 56 Table 6 Scarce Vc54 Rare & Scarce taxa recorded between 2000 & 2017 57 - 59 Table 7 Rare 60 - 61 Table 8 Scarce Natives & Archaeophytes extinct & thought to be extinct in Vc54 62 - 64 Table 9 Extinct Plant list for Vice-county 54, North Lincolnshire The main table details all the Vascular Plant & Stonewort taxa with records on the MapMate botanical database for Vc54 at the end of January 2017. The table comprises: Column 1 Taxon and Authority 2 Common Name 3 Total number of records for the taxon on the database at 31/01/2017 4 Year of first record 5 Year of latest record 6 Number of hectads with records before 1/01/2000 7 Number of hectads with records between 1/01/2000 & 31/01/2017 8 Number of tetrads with records between 1/01/2000 & 31/01/2017 9 Comment & Conservation status of the taxon in Vc54 10 Conservation status of the taxon in the UK A hectad is a 10km. -
Native Plants of Sydney Harbour National Park: Historical Records and Species Lists, and Their Value for Conservation Monitoring
Native plants of Sydney Harbour National Park: historical records and species lists, and their value for conservation monitoring Doug Benson National Herbarium of New South Wales, Royal Botanic Gardens, Mrs Macquaries Rd, Sydney 2000 AUSTRALIA [email protected] Abstract: Sydney Harbour National Park (lat 33° 53’S; long 151° 13’E), protects significant vegetation on the harbour foreshores close to Sydney City CBD; its floristic abundance and landscape beauty has been acknowledged since the writings of the First Fleet in 1788. Surprisingly, although historical plant collections were made as early as1802, and localised surveys have listed species for parts of the Park since the 1960s, a detailed survey of the flora of whole Park is still needed. This paper provides the first definitive list of the c.400 native flora species for Sydney Harbour National Park (total area 390 ha) showing occurrence on the seven terrestrial sub-regions or precincts (North Head, South Head, Dobroyd Head, Middle Head, Chowder Head, Bradleys Head and Nielsen Park). The list is based on historical species lists, records from the NSW Office of Environment and Heritage (formerly Dept of Environment, Climate Change and Water) Atlas, National Herbarium of New South Wales specimen details, and some additional fieldwork. 131 species have only been recorded from a single precinct site and many are not substantiated with a recent herbarium specimen (though there are historical specimens from the general area for many). Species reported in the sources but for which no current or historic specimen exists are listed separately as being of questionable/non-local status. -
The 1770 Landscape of Botany Bay, the Plants Collected by Banks and Solander and Rehabilitation of Natural Vegetation at Kurnell
View metadata, citation and similar papers at core.ac.uk brought to you by CORE provided by Hochschulschriftenserver - Universität Frankfurt am Main Backdrop to encounter: the 1770 landscape of Botany Bay, the plants collected by Banks and Solander and rehabilitation of natural vegetation at Kurnell Doug Benson1 and Georgina Eldershaw2 1Botanic Gardens Trust, Mrs Macquaries Rd Sydney 2000 AUSTRALIA email [email protected] 2Parks & Wildlife Division, Dept of Environment and Conservation (NSW), PO Box 375 Kurnell NSW 2231 AUSTRALIA email [email protected] Abstract: The first scientific observations on the flora of eastern Australia were made at Botany Bay in April–May 1770. We discuss the landscapes of Botany Bay and particularly of the historic landing place at Kurnell (lat 34˚ 00’ S, long 151˚ 13’ E) (about 16 km south of central Sydney), as described in the journals of Lieutenant James Cook and Joseph Banks on the Endeavour voyage in 1770. We list 132 plant species that were collected at Botany Bay by Banks and Daniel Solander, the first scientific collections of Australian flora. The list is based on a critical assessment of unpublished lists compiled by authors who had access to the collection of the British Museum (now Natural History Museum), together with species from material at National Herbarium of New South Wales that has not been previously available. The list includes Bidens pilosa which has been previously regarded as an introduced species. In 1770 the Europeans set foot on Aboriginal land of the Dharawal people. Since that time the landscape has been altered in response to a succession of different land-uses; farming and grazing, commemorative tree planting, parkland planting, and pleasure ground and tourist visitation. -
Ecology of Pyrmont Peninsula 1788 - 2008
Transformations: Ecology of Pyrmont peninsula 1788 - 2008 John Broadbent Transformations: Ecology of Pyrmont peninsula 1788 - 2008 John Broadbent Sydney, 2010. Ecology of Pyrmont peninsula iii Executive summary City Council’s ‘Sustainable Sydney 2030’ initiative ‘is a vision for the sustainable development of the City for the next 20 years and beyond’. It has a largely anthropocentric basis, that is ‘viewing and interpreting everything in terms of human experience and values’(Macquarie Dictionary, 2005). The perspective taken here is that Council’s initiative, vital though it is, should be underpinned by an ecocentric ethic to succeed. This latter was defined by Aldo Leopold in 1949, 60 years ago, as ‘a philosophy that recognizes[sic] that the ecosphere, rather than any individual organism[notably humans] is the source and support of all life and as such advises a holistic and eco-centric approach to government, industry, and individual’(http://dictionary.babylon.com). Some relevant considerations are set out in Part 1: General Introduction. In this report, Pyrmont peninsula - that is the communities of Pyrmont and Ultimo – is considered as a microcosm of the City of Sydney, indeed of urban areas globally. An extensive series of early views of the peninsula are presented to help the reader better visualise this place as it was early in European settlement (Part 2: Early views of Pyrmont peninsula). The physical geography of Pyrmont peninsula has been transformed since European settlement, and Part 3: Physical geography of Pyrmont peninsula describes the geology, soils, topography, shoreline and drainage as they would most likely have appeared to the first Europeans to set foot there. -
Newsletter No.11
AUSTRALIAN NATIVE PLANTS SOCIETY (AUSTRALIA) 1! WARATAH & FLANNEL FLOWER STUDY GROUP NO.11 JULY 2016 ISSN 1838-9082 NEWSLETTER Leader: Maria Hitchcock Welcome to any 16 Hitchcock Lane new readers! In this issue. Armidale NSW 2350 Ph. 02 6775 1139 Why not join us? Maria writes: p. 2 [email protected] It’s free! From the members p. 3 Checklist of Telopea species p. 8/9 Just send an and varieties email. Checklist of Actinotus species p. 1o and varieties New Website Bookmark it today! waratahflannelflowersg.weebly.com Past Newsletters are available here http://waratahflannelflowersg.weebly.com/ Telopea ‘Brimstone Blush’ newsletters.html Image: www.homelife.com.au The Waratah and Flannel Flower Study Group is afliated with the Australian Native Plants Society (Australia) - ANPSA This is an electronic only group. Newsletters are sent out twice a year (electronic only). Membership is free to individuals and APS (SGAP) groups. There is no deadline for newsletter contributions - send them anytime, sooner rather than later. AUSTRALIAN NATIVE PLANTS SOCIETY (AUSTRALIA) 2! WARATAH & FLANNEL FLOWER STUDY GROUP NO.11 JULY 2016 Maria writes: time visiting David Tranter whose Alloxylon grove is a sight to behold. I’m writing this from the balcony of an David took us to Robertson Railway station elevated surf shack in the Nambucca Heads where there is a wonderful collection of Caravan Park. Where else can you feel like a waratahs. We must return when they are in millionaire for the cost of less than $100 a flower. David also showed me a new way of night? We are so blessed in this country.