Nuclear Localisation and Function of the G Protein-Coupled Receptor Kinase 4 Subfamily
Total Page:16
File Type:pdf, Size:1020Kb
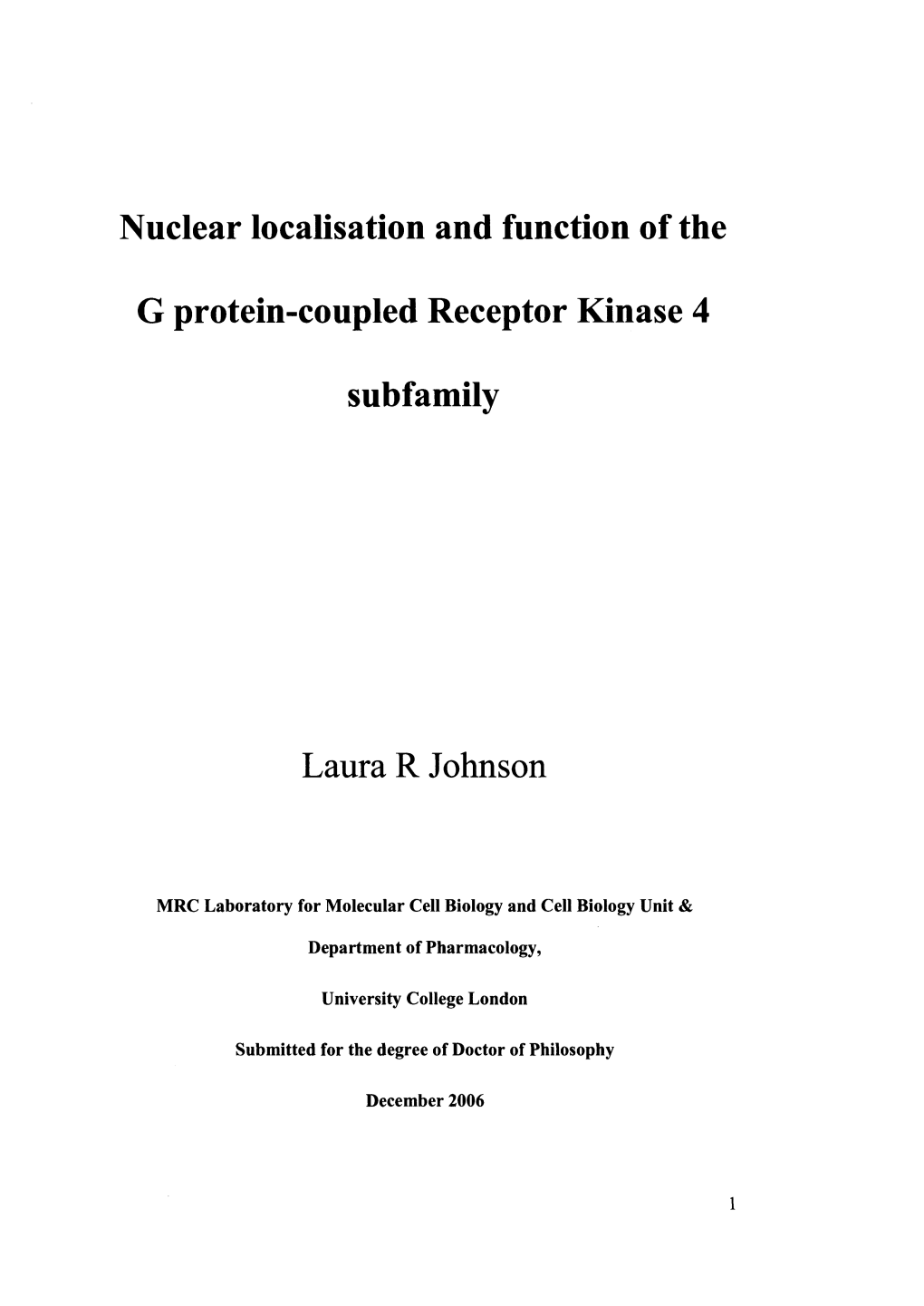
Load more
Recommended publications
-
Cytogenomic SNP Microarray - Fetal ARUP Test Code 2002366 Maternal Contamination Study Fetal Spec Fetal Cells
Patient Report |FINAL Client: Example Client ABC123 Patient: Patient, Example 123 Test Drive Salt Lake City, UT 84108 DOB 2/13/1987 UNITED STATES Gender: Female Patient Identifiers: 01234567890ABCD, 012345 Physician: Doctor, Example Visit Number (FIN): 01234567890ABCD Collection Date: 00/00/0000 00:00 Cytogenomic SNP Microarray - Fetal ARUP test code 2002366 Maternal Contamination Study Fetal Spec Fetal Cells Single fetal genotype present; no maternal cells present. Fetal and maternal samples were tested using STR markers to rule out maternal cell contamination. This result has been reviewed and approved by Maternal Specimen Yes Cytogenomic SNP Microarray - Fetal Abnormal * (Ref Interval: Normal) Test Performed: Cytogenomic SNP Microarray- Fetal (ARRAY FE) Specimen Type: Direct (uncultured) villi Indication for Testing: Patient with 46,XX,t(4;13)(p16.3;q12) (Quest: EN935475D) ----------------------------------------------------------------- ----- RESULT SUMMARY Abnormal Microarray Result (Male) Unbalanced Translocation Involving Chromosomes 4 and 13 Classification: Pathogenic 4p Terminal Deletion (Wolf-Hirschhorn syndrome) Copy number change: 4p16.3p16.2 loss Size: 5.1 Mb 13q Proximal Region Deletion Copy number change: 13q11q12.12 loss Size: 6.1 Mb ----------------------------------------------------------------- ----- RESULT DESCRIPTION This analysis showed a terminal deletion (1 copy present) involving chromosome 4 within 4p16.3p16.2 and a proximal interstitial deletion (1 copy present) involving chromosome 13 within 13q11q12.12. This -
A Computational Approach for Defining a Signature of Β-Cell Golgi Stress in Diabetes Mellitus
Page 1 of 781 Diabetes A Computational Approach for Defining a Signature of β-Cell Golgi Stress in Diabetes Mellitus Robert N. Bone1,6,7, Olufunmilola Oyebamiji2, Sayali Talware2, Sharmila Selvaraj2, Preethi Krishnan3,6, Farooq Syed1,6,7, Huanmei Wu2, Carmella Evans-Molina 1,3,4,5,6,7,8* Departments of 1Pediatrics, 3Medicine, 4Anatomy, Cell Biology & Physiology, 5Biochemistry & Molecular Biology, the 6Center for Diabetes & Metabolic Diseases, and the 7Herman B. Wells Center for Pediatric Research, Indiana University School of Medicine, Indianapolis, IN 46202; 2Department of BioHealth Informatics, Indiana University-Purdue University Indianapolis, Indianapolis, IN, 46202; 8Roudebush VA Medical Center, Indianapolis, IN 46202. *Corresponding Author(s): Carmella Evans-Molina, MD, PhD ([email protected]) Indiana University School of Medicine, 635 Barnhill Drive, MS 2031A, Indianapolis, IN 46202, Telephone: (317) 274-4145, Fax (317) 274-4107 Running Title: Golgi Stress Response in Diabetes Word Count: 4358 Number of Figures: 6 Keywords: Golgi apparatus stress, Islets, β cell, Type 1 diabetes, Type 2 diabetes 1 Diabetes Publish Ahead of Print, published online August 20, 2020 Diabetes Page 2 of 781 ABSTRACT The Golgi apparatus (GA) is an important site of insulin processing and granule maturation, but whether GA organelle dysfunction and GA stress are present in the diabetic β-cell has not been tested. We utilized an informatics-based approach to develop a transcriptional signature of β-cell GA stress using existing RNA sequencing and microarray datasets generated using human islets from donors with diabetes and islets where type 1(T1D) and type 2 diabetes (T2D) had been modeled ex vivo. To narrow our results to GA-specific genes, we applied a filter set of 1,030 genes accepted as GA associated. -
Comprehensive Assessment of the Association of WNK4
OPEN Comprehensive Assessment of the SUBJECT AREAS: Association of WNK4 Polymorphisms GENETICS RESEARCH RENOVASCULAR HYPERTENSION with Hypertension: Evidence from a EPIDEMIOLOGY Meta-Analysis Received Xiao-gang Guo1, Jie Ding1, Hui Xu1,2, Tian-ming Xuan1, Wei-quan Jin1, Xiang Yin1, Yun-peng Shang1, 22 April 2014 Fu-rong Zhang1, Jian-hua Zhu1 & Liang-rong Zheng1 Accepted 15 September 2014 1Department of Cardiology, the First Affiliated Hospital, School of Medicine, Zhejiang University, Hangzhou 310003, China, Published 2Xiuzhou District, Gaozhao Street Community Health Service Center, Jiaxing 314031, China. 30 September 2014 The relationship between with-no-lysine [K] kinase 4 (WNK4) gene polymorphisms and hypertension has been widely investigated, However, the studies yielded contradictory results. To evaluate these inconclusive Correspondence and findings comprehensively, we therefore performed a meta-analysis. Ten articles encompassing 16 requests for materials independent case-control studies with 6089 hypertensive cases and 4881 normotensive controls were should be addressed to selected for this meta-analysis. Four WNK4 gene polymorphisms were identified (G1155942T, G1156666A, X.-G.G. (gxg22222@ T1155547C, and C6749T). The results showed statistically significant associations of G1155942T polymorphism (allelic genetic model: odds ration or OR51.62, 95% confidence interval or CI: 1.11–2.38, zju.edu.cn) P50.01; dominant model: OR51.85, 95% CI: 1.07–3.19, P50.03) and C6749T polymorphism (allele contrast: OR52.04, 95% CI: 1.60–2.59, P,0.01; dominant model: OR52.04, 95%CI: 1.59–2.62, P,0.01; and homozygous model: OR55.01, 95% CI: 1.29–19.54, P50.02) with hypertension risk. However, neither C1155547T nor G1156666A was associated significantly with hypertension susceptibility. -
The Wild Species Genome Ancestry of Domestic Chickens Short Title: Chicken Genome Ancestry
Supplementary Materials Full title: The wild species genome ancestry of domestic chickens Short title: Chicken genome ancestry Raman Akinyanju Lawal1,2*#, Simon H. Martin3,4, Koen Vanmechelen5, Addie Vereijken6, Pradeepa Silva7, Raed Mahmod Al-Atiyat8, Riyadh Salah Aljumaah9, Joram M. Mwacharo10, Dong-Dong Wu11,12, Ya-Ping Zhang11,12, Paul M. Hocking13†, Jacqueline Smith13, David Wragg14 & Olivier Hanotte1, 14,15* 1Cells, Organisms and Molecular Genetics, School of Life Sciences, University of Nottingham, NG7 2RD, Nottingham, United Kingdom 2,#The Jackson Laboratory, 600 Main Street, Bar Harbor, ME 04609, USA 3Institute of Evolutionary Biology, University of Edinburgh, EH9 3FL, Edinburgh, United Kingdom 4Department of Zoology, University of Cambridge, CB2 3EJ, Cambridge, United Kingdom 5Open University of Diversity - Mouth Foundation, Hasselt, Belgium 6Hendrix Genetics, Technology and Service B.V., P.O. Box 114, 5830, AC, Boxmeer, The Netherlands 7Department of Animal Sciences, Faculty of Agriculture, University of Peradeniya, Sri Lanka 8Genetics and Biotechnology, Animal Science Department, Agriculture Faculty, Mutah University, Karak, Jordan 9Department of Animal Production, King Saud University, Saudi Arabia 10Small Ruminant Genomics, International Centre for Agricultural Research in the Dry Areas (ICARDA), P.O. Box 5689, ILRI-Ethiopia Campus, Addis Ababa, Ethiopia 11Center for Excellence in Animal Evolution and Genetics, Chinese Academy of Sciences, 650223 Kunming, China 12State Key Laboratory of Genetic Resources and Evolution, Kunming Institute of Zoology, Chinese Academy of Sciences, 650223 Kunming, China. 13The Roslin Institute and Royal (Dick) School of Veterinary Studies, University of Edinburgh, Easter Bush Campus, Midlothian, EH25 9RG, UK 14Centre for Tropical Livestock Genetics and Health, The Roslin Institute, EH25 9RG, Edinburgh, UK 15LiveGene, International Livestock Research Institute (ILRI), P. -
Comprehensive Assessment of Indian Variations in the Druggable Kinome Landscape Highlights Distinct Insights at the Sequence, Structure and Pharmacogenomic Stratum
SUPPLEMENTARY MATERIAL Comprehensive assessment of Indian variations in the druggable kinome landscape highlights distinct insights at the sequence, structure and pharmacogenomic stratum Gayatri Panda1‡, Neha Mishra1‡, Disha Sharma2,3, Rahul C. Bhoyar3, Abhinav Jain2,3, Mohamed Imran2,3, Vigneshwar Senthilvel2,3, Mohit Kumar Divakar2,3, Anushree Mishra3, Priyanka Banerjee4, Sridhar Sivasubbu2,3, Vinod Scaria2,3, Arjun Ray1* 1 Department of Computational Biology, Indraprastha Institute of Information Technology, Okhla, India. 2 Academy of Scientific and Innovative Research (AcSIR), Ghaziabad, India. 3 CSIR-Institute of Genomics and Integrative Biology, Mathura Road, Delhi-110020, India. 4 Institute for Physiology, Charite-University of Medicine, Berlin, 10115 Berlin, Germany. ‡These authors contributed equally to this work. * [email protected] TABLE OF CONTENTS Name Title Supplemental_Figure_S1 Fauchere and Pliska hydrophobicity scale for variations in structure data Supplemental_Figure_S2 Phenotypic drug-drug correlogram Supplemental_Table_S1 545 kinase coding genes used in the study Supplemental_Table_S2 Classes and count of kinase coding genes Supplemental_Table_S3 Allele frequency Indian v/s other populations from 1000 genome data(1000g2015). Supplemental_Table_S4 IndiGen Structure Data- consisting of 12 genes and their 22 variants Supplemental_Table_S5 Genes, PDB ids, mutations in IndiGen data and associated drugs (FDA approved) Supplemental_Table_S6 Data used for docking and binding pocket similarity analysis Supplemental_Table_S7 -
G Protein-Coupled Receptor Kinase 4 Gene Variants in Human Essential Hypertension
G protein-coupled receptor kinase 4 gene variants in human essential hypertension Robin A. Felder*, Hironobu Sanada*†, Jing Xu‡, Pei-Ying Yu‡, Zheng Wang‡, Hidetsuna Watanabe*, Laureano D. Asico*, Wei Wang‡, Shaopeng Zheng‡, Ikuyo Yamaguchi‡, Scott M. Williams§, James Gainer¶, Nancy J. Brown¶, Debra Hazen-Martinʈ, Lee-Jun C. Wong**, Jean E. Robillard††, Robert M. Carey‡‡, Gilbert M. Eisner‡, and Pedro A. Jose‡ *Department of Pathology, University of Virginia Health Sciences Center, Charlottesville, VA 22908; ‡Department of Pediatrics and Physiology and Biophysics, Georgetown University Medical Center, Washington, DC 20007; §Department of Microbiology, Meharry Medical College, Nashville, TN 37208; ¶Department of Medicine and Pharmacology, Vanderbilt University Medical Center, Nashville, TN 37232; ʈDepartment of Pathology, Medical University of South Carolina, Charleston, SC 29403; **Institute for Molecular and Human Genetics, Georgetown University Medical Center, Washington, DC 20007; ††Department of Pediatrics, University of Michigan College of Medicine, Ann Arbor, MI 48109; and ‡‡Department of Medicine, University of Virginia Health Sciences Center, Charlottesville, VA 22908 Communicated by Maria Iandolo New, Weill Medical College of Cornell University, New York, NY, December 21, 2001 (received for review August 10, 2001) Essential hypertension has a heritability as high as 30–50%, but its abnormal renal sodium transporters (3, 8, 13, 17). Also, the genetic cause(s) has not been determined despite intensive inves- coding region of the D1 receptor is unchanged in hypertensive tigation. The renal dopaminergic system exerts a pivotal role in subjects (16), as well as in rodents with genetic hypertension maintaining fluid and electrolyte balance and participates in the (unpublished studies). pathogenesis of genetic hypertension. -
GRK4 Protein Recombinant Human Protein Expressed in Sf9 Cells
Catalog # Aliquot Size G02-31G-20 20 µg G02-31G-50 50 µg GRK4 Protein Recombinant human protein expressed in Sf9 cells Catalog # G02-31G Lot # P1749-5 Product Description Purity Recombinant human GRK4 (140-end) was expressed by baculovirus in Sf9 insect cells using an N-terminal GST tag. The gene accession number for GRK4 is BC117320. The purity of GRK4 was determined to be >70% by densitometry, GRK4 Gene Aliases approx. MW 75kDa. GPRK2L, GPRK4, GRK4a, IT11 Formulation Recombinant protein stored in 50mM Tris-HCl, pH 7.5, 150mM NaCl, 10mM glutathione, 0.1mM EDTA, 0.25mM DTT, 0.1mM PMSF, 25% glycerol. Storage and Stability o Store product at –70 C. For optimal storage, aliquot target into smaller quantities after centrifugation and store at recommended temperature. For most favorable performance, avoid repeated handling and multiple freeze/thaw cycles. Scientific Background GRK4 or G protein-coupled receptor kinase 4 is a member of the guanine nucleotide-binding protein (G protein)-coupled receptor kinase subfamily of the Ser/Thr protein kinase family which phosphorylates the activated forms of G protein-coupled receptors thus initiating its deactivation and it also play an important role in receptor desensitization. GRK4 protein has kinase activity GRK4 Protein and that it interacts with and is inhibitable by calmodulin Recombinant human protein expressed in Sf9 cells (1).GRK4 has been linked to both genetic and acquired Catalog # hypertension. GRK4 is highly expressed in testis (2). G02-31G Lot # P1749-5 References Purity >70% Concentration 0.05 µg/µl Stability 1yr at –70oC from date of shipment 1. -
Screening Differentially Expressed Genes of Pancreatic Cancer
Xu et al. BMC Cancer (2020) 20:298 https://doi.org/10.1186/s12885-020-06722-7 RESEARCH ARTICLE Open Access Screening differentially expressed genes of pancreatic cancer between Mongolian and Han people using bioinformatics technology Jiasheng Xu1†, Kaili Liao2†, Zhonghua Fu3* and Zhenfang Xiong1* Abstract Background: To screen and analyze differentially expressed genes in pancreatic carcinoma tissues taken from Mongolian and Han patients by Affymetrix Genechip. Methods: Pancreatic ductal cell carcinoma tissues were collected from the Mongolian and Han patients undergoing resection in the Second Affiliated Hospital of Nanchang University from March 2015 to May 2018 and the total RNA was extracted. Differentially expressed genes were selected from the total RNA qualified by Nanodrop 2000 and Agilent 2100 using Affymetrix and a cartogram was drawn; The gene ontology (GO) analysis and Pathway analysis were used for the collection and analysis of biological information of these differentially expressed genes. Finally, some differentially expressed genes were verified by real-time PCR. Results: Through the microarray analysis of gene expression, 970 differentially expressed genes were detected by comparing pancreatic cancer tissue samples between Mongolian and Han patients. A total of 257 genes were significantly up-regulated in pancreatic cancer tissue samples in Mongolian patients; while a total of 713 genes were down-regulated. In the Gene Ontology database, 815 differentially expressed genes were identified with clear GO classification, and CPB1 gene showed the highest increase in expression level (multiple difference: 31.76). The pathway analysis detected 28 signaling pathways that included these differentially expressed genes, involving a total of 178 genes. Among these pathways, the enrichment of differentially expressed genes in the FAK signaling pathway was the strongest and COL11A1 gene showed the highest multiple difference (multiple difference: 5.02). -
Supplementary Table S1. Kinase Selectivity of MPI-0479605. (A)
A B In-house kinase profiling Invitrogen SelectScreen Profiling % % % Kinase IC50 ( M) Kinase IC50 ( M) inhibition inhibition inhibition AKT3 >5 IKK-epsilon >5 Kinase at 500 nM Kinase at 500 nM Kinase at 500 nM ALK 0.26 INSR 0.38 ABL1 7 GRK6 -7 PAK1 4 AUR-A >5 JAK1 >5 GRK2 4 HCK 7 PASK 6 AUR-B >5 JNK1 0.11 AKT1 2 HIPK2 9 PHKG1 8 AUR-C >5 MEK1 >5 AXL 6 HIPK4 -6 PRKCB1 -2 B-RAF 3.2 MST4 >5 BMX 14 IGF1R 37 PRKCE 0 CDK1 >5 NEK2 >5 BRSK1 -9 IRAK4 0 PRKCG 3 CDK2 >5 PDK1 >5 CAMK2D -1 ITK 9 PRKCI -2 CDK6 >5 PKA >5 CDC42 BPA 0 LCK 29 PRKCN 6 CHK1 >5 PKC-delta >5 CDK5/p25 -1 LIMK1 17 PRKCZ -5 CHK2 >5 PLK1 >5 CSF1R 82 MAP2K1 11 PRKG2 2 C-MET >5 PLK4 3.3 CSK 1 MAP2K6 2 PTK6 15 C-SRC >5 ROCK2 >5 CSNK1D 10 MAP3K3 17 RET 11 DYRK2 >5 RSK2 >5 CSNK1G1 -1 MAP3K5 -1 RPS6KA2 23 ERK2 3.9 STK33 1.1 CSNK2A2 33 MAP3K8 12 RPS6KA5 2 FAK1 2.7 TAK1 >5 DCAMKL2 2 MAPK1 0 SGK2 4 FER 0.59 TAO1 >5 DYRK1B 1 MAPK11 2 SNF1LK2 3 FLT3 0.080 TBK1 >5 EEF2K 2 MAPK12 5 SRPK2 -3 HGK >5 TRKB >5 EGFR 14 MAPK3 -2 STK22B 11 IKK-alpha >5 YES1 >5 EPHA1 6 MAPK9 61 STK23 -1 IKK-beta >5 EPHA4 -10 MAPKAPK2 9 STK24 -2 EPHB1 -33 MARK4 3 STK25 0 ERBB2 5 MELK 6 TEK 6 FGFR1 15 MERTK 7 TYK2 10 FGR 17 MKNK1 2 TYRO3 -2 FLT4 15 MST1R 3 ZAP70 5 FRAP1 7 NEK1 1 GRK4 3 NEK4 -6 Supplementary Table S1. -
Hepatic Malignancy in an Infant with Wolf--Hirschhorn Syndrome
Fetal and Pediatric Pathology ISSN: 1551-3815 (Print) 1551-3823 (Online) Journal homepage: https://www.tandfonline.com/loi/ipdp20 Hepatic Malignancy in an Infant with Wolf–Hirschhorn Syndrome Sara Rutter, Raffaella A Morotti, Steven Peterec & Patrick G. Gallagher To cite this article: Sara Rutter, Raffaella A Morotti, Steven Peterec & Patrick G. Gallagher (2017) Hepatic Malignancy in an Infant with Wolf–Hirschhorn Syndrome, Fetal and Pediatric Pathology, 36:3, 256-262, DOI: 10.1080/15513815.2017.1293201 To link to this article: https://doi.org/10.1080/15513815.2017.1293201 Published online: 07 Mar 2017. Submit your article to this journal Article views: 90 View related articles View Crossmark data Full Terms & Conditions of access and use can be found at https://www.tandfonline.com/action/journalInformation?journalCode=ipdp20 FETAL AND PEDIATRIC PATHOLOGY ,VOL.,NO.,– http://dx.doi.org/./.. CASE REPORT Hepatic Malignancy in an Infant with Wolf–Hirschhorn Syndrome Sara Ruttera, Raffaella A Morottia,b, Steven Peterecb, and Patrick G. Gallaghera,b,c aDepartment of Pathology, Yale University School of Medicine, New Haven, Connecticut, USA; bDepartment of Pediatrics, Yale University School of Medicine, New Haven, Connecticut, USA; cDepartment of Genetics, Yale University School of Medicine, New Haven, Connecticut, USA ABSTRACT ARTICLE HISTORY Introduction: Wolf–Hirschhorn syndrome (WHS) is a contiguous gene Received November syndrome involving deletions of the chromosome 4p16 region asso- Revised January ciated with growth failure, characteristic craniofacial abnormalities, Accepted January cardiac defects, and seizures. Case Report: This report describes a KEYWORDS six-month-old girl with WHS with growth failure and typical craniofacial Liver; malignancy; neonate; features who died of complex congenital heart disease. -
A Loss-Of-Function Genetic Screening Identifies Novel Mediators of Thyroid Cancer Cell Viability
www.impactjournals.com/oncotarget/ Oncotarget, Vol. 7, No. 19 A loss-of-function genetic screening identifies novel mediators of thyroid cancer cell viability Maria Carmela Cantisani1, Alessia Parascandolo2, Merja Perälä3,4, Chiara Allocca2, Vidal Fey3,4, Niko Sahlberg3,4, Francesco Merolla5, Fulvio Basolo6, Mikko O. Laukkanen1, Olli Pekka Kallioniemi7, Massimo Santoro2,8, Maria Domenica Castellone8 1IRCCS SDN, Naples, Italy 2Dipartimento di Medicina Molecolare e Biotecnologie Mediche, Universita’ Federico II, Naples, Italy 3Medical Biotechnology, VTT Technical Research Centre of Finland, Turku, Finland 4Center for Biotechnology, University of Turku, Turku, Finland 5Dipartimento di Scienze Biomediche Avanzate, Università Federico II, Naples, Italy 6Division of Pathology, Department of Surgery, University of Pisa, Pisa, Italy 7FIMM-Institute for Molecular Medicine Finland, University of Helsinki, Helsinki, Finland 8Istituto di Endocrinologia ed Oncologia Sperimentale “G. Salvatore” (IEOS), C.N.R., Naples, Italy Correspondence to: Maria Domenica Castellone, e-mail: [email protected] Keywords: kinases, screening, siRNA, thyroid carcinoma Received: October 01, 2015 Accepted: March 02, 2016 Published: April 4, 2016 ABSTRACT RET, BRAF and other protein kinases have been identified as major molecular players in thyroid cancer. To identify novel kinases required for the viability of thyroid carcinoma cells, we performed a RNA interference screening in the RET/PTC1(CCDC6- RET)-positive papillary thyroid cancer cell line TPC1 using a library of synthetic small interfering RNAs (siRNAs) targeting the human kinome and related proteins. We identified 14 hits whose silencing was able to significantly reduce the viability and the proliferation of TPC1 cells; most of them were active also in BRAF-mutant BCPAP (papillary thyroid cancer) and 8505C (anaplastic thyroid cancer) and in RAS-mutant CAL62 (anaplastic thyroid cancer) cells. -
Gene Symbol Accession Alias/Prev Symbol Official Full Name AAK1 NM 014911.2 KIAA1048, Dkfzp686k16132 AP2 Associated Kinase 1
Gene Symbol Accession Alias/Prev Symbol Official Full Name AAK1 NM_014911.2 KIAA1048, DKFZp686K16132 AP2 associated kinase 1 (AAK1) AATK NM_001080395.2 AATYK, AATYK1, KIAA0641, LMR1, LMTK1, p35BP apoptosis-associated tyrosine kinase (AATK) ABL1 NM_007313.2 ABL, JTK7, c-ABL, p150 v-abl Abelson murine leukemia viral oncogene homolog 1 (ABL1) ABL2 NM_007314.3 ABLL, ARG v-abl Abelson murine leukemia viral oncogene homolog 2 (arg, Abelson-related gene) (ABL2) ACVR1 NM_001105.2 ACVRLK2, SKR1, ALK2, ACVR1A activin A receptor ACVR1B NM_004302.3 ACVRLK4, ALK4, SKR2, ActRIB activin A receptor, type IB (ACVR1B) ACVR1C NM_145259.2 ACVRLK7, ALK7 activin A receptor, type IC (ACVR1C) ACVR2A NM_001616.3 ACVR2, ACTRII activin A receptor ACVR2B NM_001106.2 ActR-IIB activin A receptor ACVRL1 NM_000020.1 ACVRLK1, ORW2, HHT2, ALK1, HHT activin A receptor type II-like 1 (ACVRL1) ADCK1 NM_020421.2 FLJ39600 aarF domain containing kinase 1 (ADCK1) ADCK2 NM_052853.3 MGC20727 aarF domain containing kinase 2 (ADCK2) ADCK3 NM_020247.3 CABC1, COQ8, SCAR9 chaperone, ABC1 activity of bc1 complex like (S. pombe) (CABC1) ADCK4 NM_024876.3 aarF domain containing kinase 4 (ADCK4) ADCK5 NM_174922.3 FLJ35454 aarF domain containing kinase 5 (ADCK5) ADRBK1 NM_001619.2 GRK2, BARK1 adrenergic, beta, receptor kinase 1 (ADRBK1) ADRBK2 NM_005160.2 GRK3, BARK2 adrenergic, beta, receptor kinase 2 (ADRBK2) AKT1 NM_001014431.1 RAC, PKB, PRKBA, AKT v-akt murine thymoma viral oncogene homolog 1 (AKT1) AKT2 NM_001626.2 v-akt murine thymoma viral oncogene homolog 2 (AKT2) AKT3 NM_181690.1